10 +Ice
Jeff Kavanaugh; Britta Jensen; and John W.F. Waldron
This chapter is a draft. Figures, glossary entries, and links need to be added.
Learning Outcomes
By the end of this section you should be able to:
- Describe the main components of the Cryosphere;
- Define ‘glacier’ and describe how glaciers are classified, and how they form and move in different thermal regimes;
- Define glacier mass balance and outline how it controls glacial advance and retreat;
- Describe how glaciers modify landscapes through erosion and deposition, and identify the erosional and depositional features produced;
- Describe periglacial landscapes, including permafrost, and how they interact with changing climate;
- Describe how sea ice forms and how it interacts with global climate.
Introduction to the Crysophere
Properties of ice
The part of the Hydrosphere that is composed of water in the solid state is known as the Crysophere (named from the ancient Greek word for ice). Ice plays an extremely important role in Earth’s climate, affecting both the atmosphere and the other, liquid parts of the Hydrosphere. The physical properties of ice, mentioned in an earlier section on water, are extremely important for these effects, and it’s worth revisiting them here.
Perhaps the most unusual property of ice is that it is less dense than liquid water. Almost all other substances become more dense when they freeze. The crystal structure of ice is exceptional because hydrogen bonds between the polar water molecules maintain a very open structure. As water cools, it starts to expand at 4°C, and as it crystallizes into ice it continues to expand down to a temperature of -20°C. The most important result of this unusual behaviour is that ice floats on water.
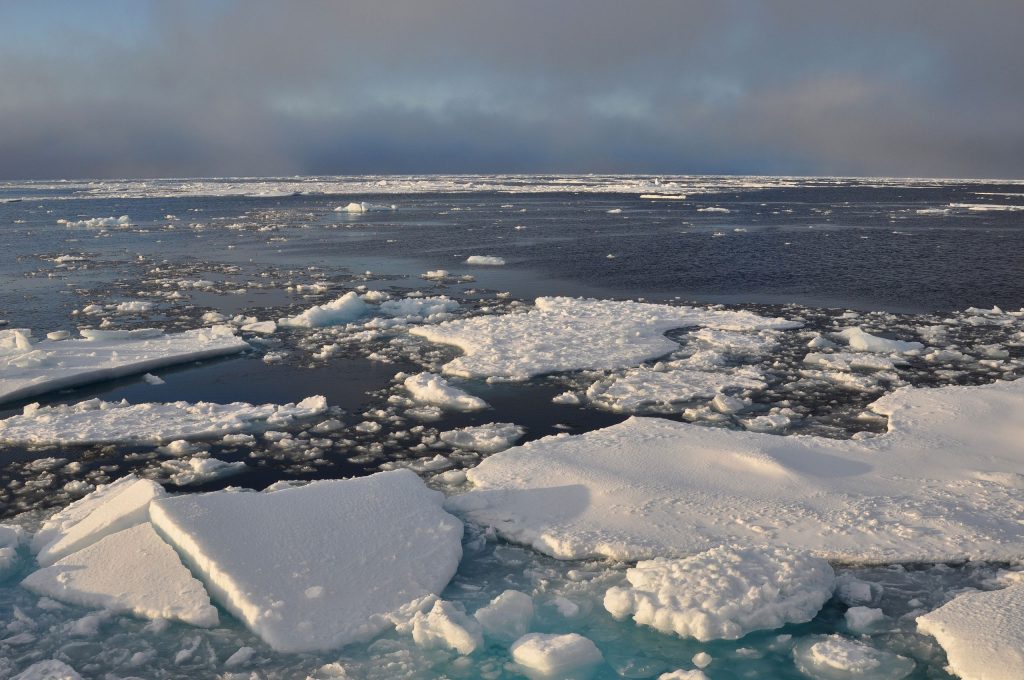
A second property of ice that is important in climate is that ice, and especially the finely crystalline form known as snow, is extremely reflective: its albedo can be above 0.8, indicating that over 80% of incoming solar energy is reflected, not absorbed. In detail, the albedo of snow is quite variable, and depends on its grain-size, the presence of impurities, and the degree of melting. However, even melting or dirty snow typically has an albedo of 0.5, making it more reflective than much of the Earth’s surface.
A third property of ice that is important for ocean circulation is that ice crystals are unable to accommodate most of the ions that are dissolved in sea-water. When sea-water partially freezes, the remaining, left-over water contains high concentrations of these ions, making it particularly dense. This phenomenon contributes to the overall thermo-haline circulation of the deep oceans, which also affects global climate.
Components of the Cryosphere
The Cryosphere comprises the parts of the Earth where water is frozen perennially (throughout the year), or freezes for at least part of the year. It includes
- Snow: ice that is crystallized in the atmosphere directly from water vapour. Snow is precipitated and covers highly variable parts of the Earth’s surface, depending on the seasons.
- Glaciers: compacted ice that flows slowly over the Earth’s surface. Glaciers cover about 10% of Earth’s land surface, and hold about 70% of Earth’s fresh water.
- Sea ice: Floating ice that accounts for two thirds of Earth’s persistent ice cover, but makes up only about 0.1% of the total ice volume because it is very thin.
- Frozen ground: Ice in the pore spaces of soil and surface sediment occurs beneath about 20% of Earth’s land surface. It is the frozen equivalent of groundwater. Where this ice persists year round, it is known as permafrost.

Snow
Distribution of snow
Snow is very unevenly distributed over the Earth’s land surface, both in space and time. Precipitation more commonly falls as snow during colder periods of the year, and at higher latitudes and higher altitudes. Because the Northern Hemisphere has a much greater land area at medium to high latitudes than does the Southern Hemisphere, snow cover varies much more significantly in the global north than it does in the global south.
<NASA animation of snow cover variations>
Snow has very high albedo – it may reflect up to about 80% of the incoming solar radiation, so snow cover produces a positive feedback: if the temperature in an area becomes low enough to produce snow, the resulting albedo may further reduce temperature. If snow accumulation exceeds melting and evaporation over a period of years, glaciers can form and grow.
In many areas where snow falls, it’s possible to mark a boundary between areas (usually at higher elevations above sea-level) that have snow year-round, and areas that lose their snow in the summer. This boundary, between areas that lose their snow in the summer and those that don’t is called the annual snowline[1].
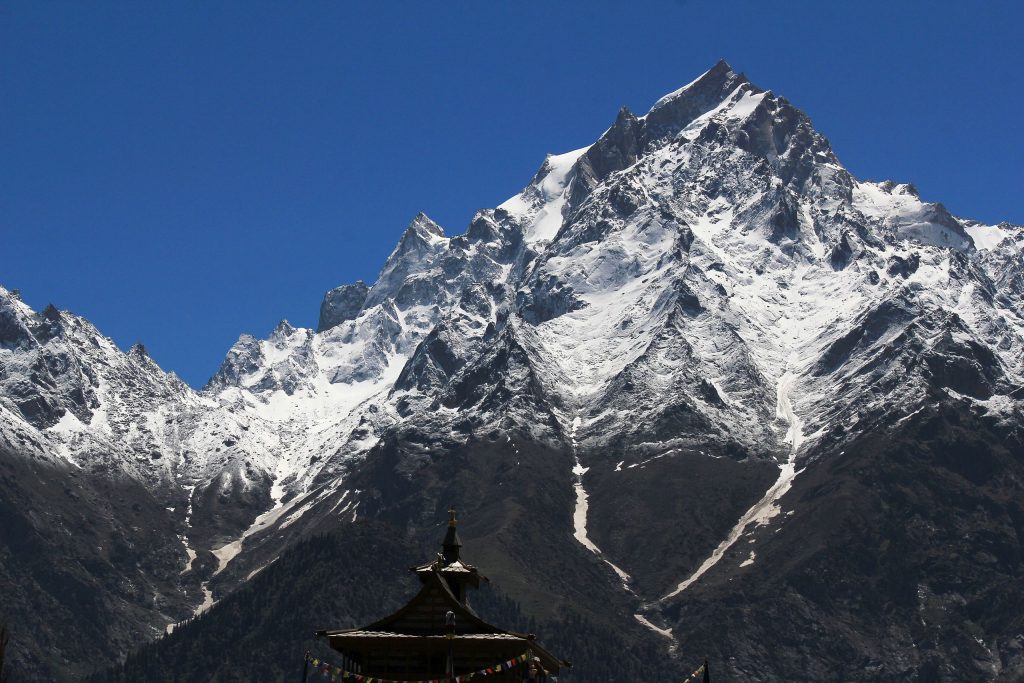
The elevation of the snowline, and therefore its shape when viewed on a map, changes from year to year, depending on winter snow accumulation and summer melting. It’s important to note that the elevation of the snowline is not just dependent on temperature.
<map showing elevation of snowline in western North America?>
The elevation of the snowline is lower:
- in polar areas where temperature is colder;
- in coastal areas where there is more moisture, and therefore more snow.
Changes in snow cover over time
The distribution of snow has changed markedly during the late 20th and 21st centuries CE. For example, between 1972 and 2020, the average North American snow cover decreased at about 4900 km2 per year. The average area covered by snow during the decade 2011–2020 was about 2% smaller than the average extent during 1972–1981. These trends continue at the time of writing (2023).
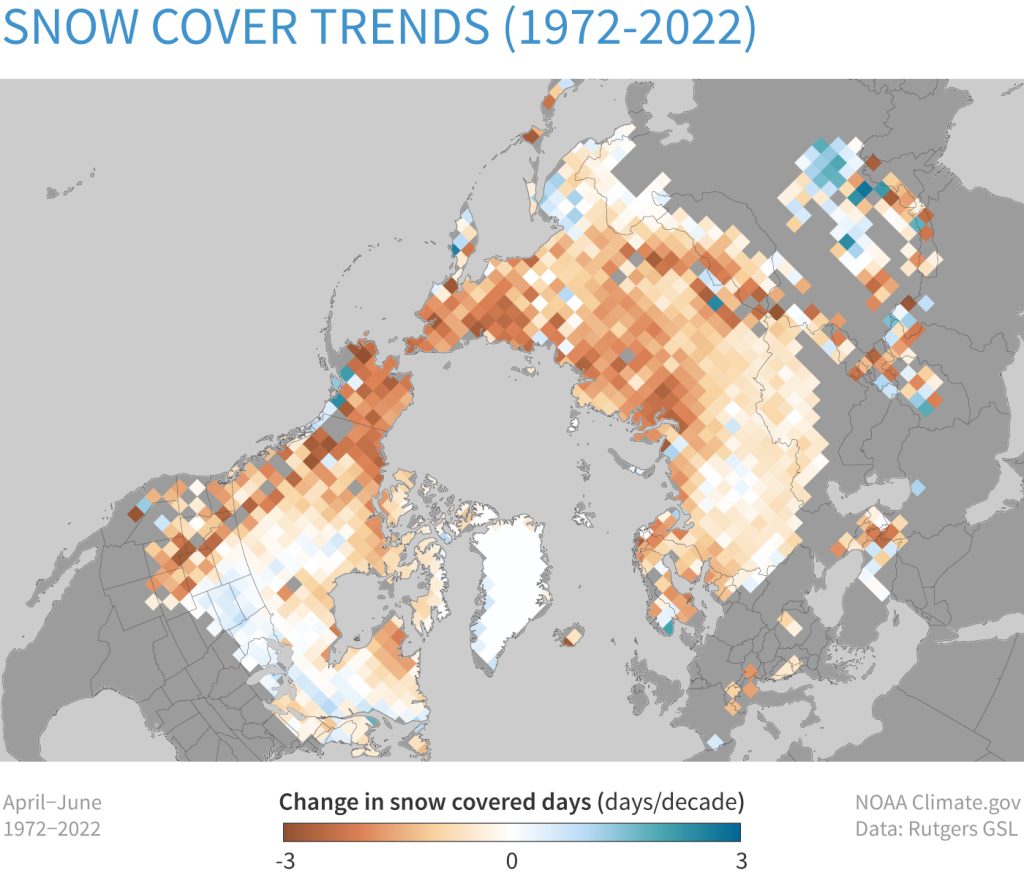
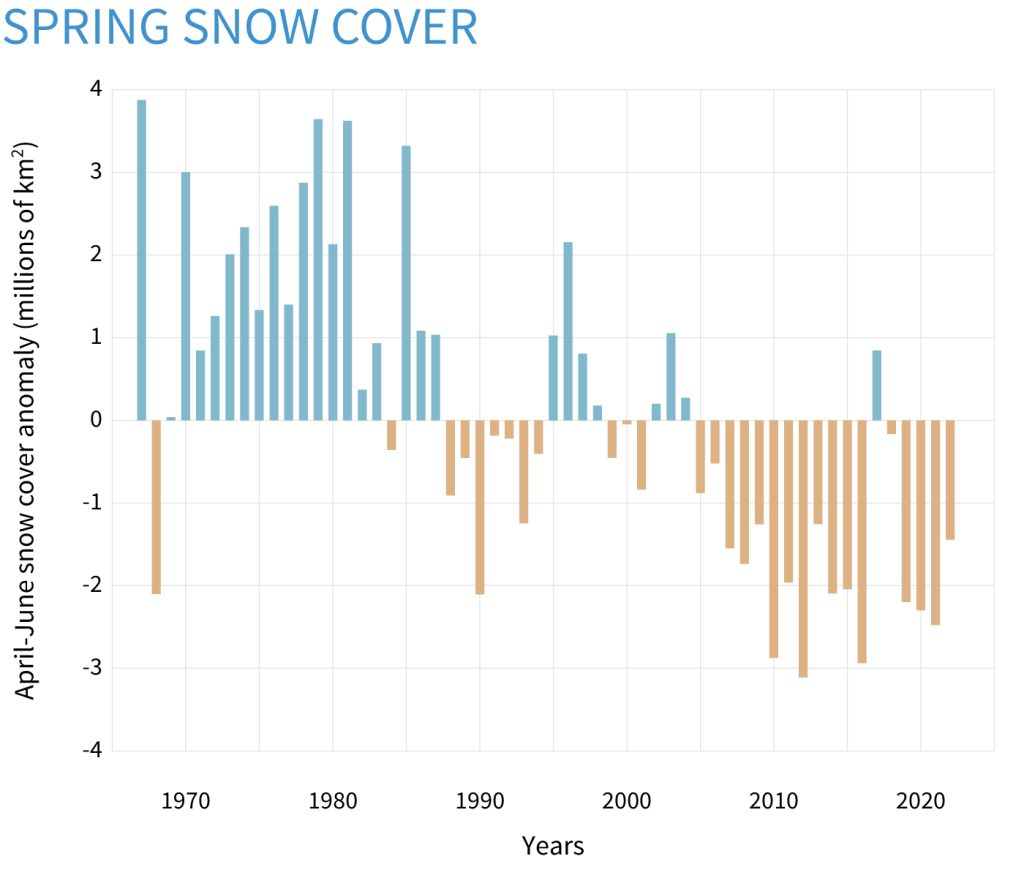
These decreases in snow cover have been most pronounced in spring and summer. As a result of these losses in spring and summer, the snow season has become shorter.
This loss of snow decreases Earth’s albedo, leading to more energy absorption, and higher temperatures, in turn leading to more snow loss, in a process of positive feedback. It is likely that in the past, during the start of ice ages, positive feedback acted in the opposite sense: a small initial temperature fall (or an increase in humidity) led to more snowfall, increased albedo, and further temperature fall.
Glaciers
What is a glacier?
Glaciers are large accumulations of perennial (year-round) ice that flow under the forces exerted by their own mass and gravity. All glaciers originate on land and move from high elevation to low elevation, although locally ice may flow up-hill as it moves over obstacles, and glacier ice may flow onto water if it reaches a lake or the sea.
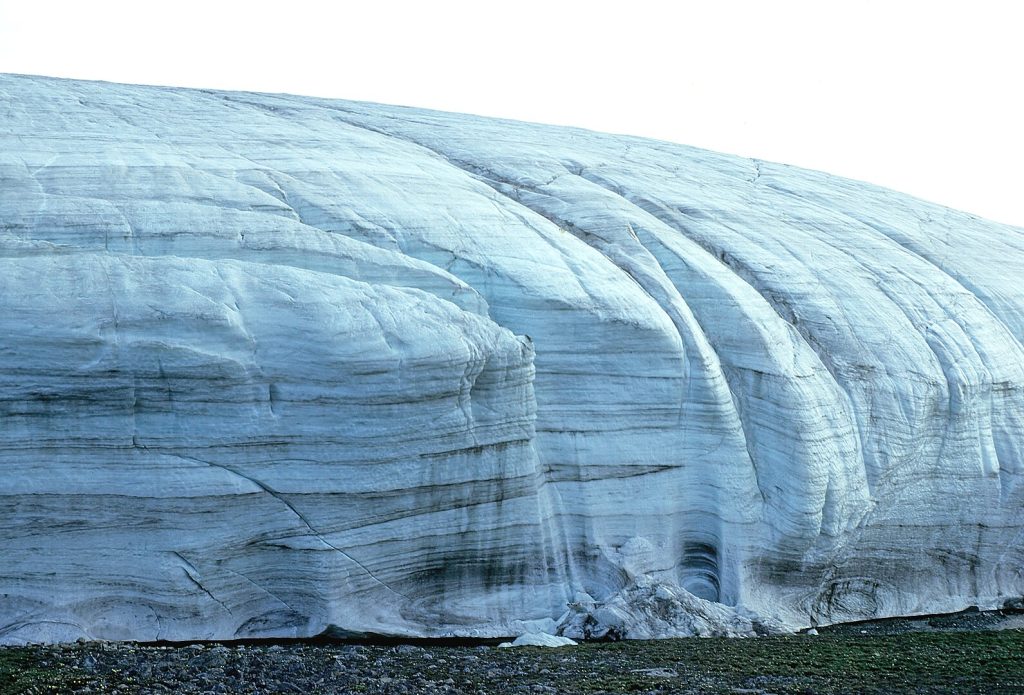
Glaciers and ice sheets currently cover about 10% of the Earth, and are major modifiers of the Earth’s surface. Because the action of ice in the past has left traces in the landscape, we know that there have been periods of time in Earth history (ice ages, also known as icehouse intervals) when ice has covered more of the land surface, and periods (greenhouse intervals) when the area covered by ice has been much less.
Glaciers may be classified in many different ways: by location, by size and shape, or by the way the base of the glacier flows over the landscape. Before classifying glaciers we will investigate the way that snow is transformed to ice and how that ice flows through a typical glacier.
Movement of ice through glaciers
How glaciers form: transformation of snow to glacier ice
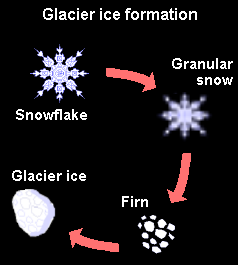
Snow passes through a number of stages as it is converted to glacier ice.
- Freshly fallen snow contains large amounts of trapped air, and as a result is much less dense than either water or solid ice. Its density typically ranges from about 50 to 150 kg/m3 (5% to 15% of the density of liquid water), although wind-packed snow may be denser, up to about 400 kg/m3.
- Summer melting causes the snowflakes to lose their fine detail and become more granular, converting the snow to a granular form known as névé.
- Névé that survives for more than one summer becomes firn. The densities of névé and firn vary from about 350 to 800 kg/m3, as pressure from overlying snow drives out the air and causes compaction.
- Firn is converted to glacier ice when the trapped air bubbles are sealed off from one another, which typically happens at a density of 830 kg/m3. Pure glacier ice, without air, has a density of 917 kg/m3, or about 92% of the density of water, although slightly higher values (up to 923 kg/m3) may occur under pressure at the base of a thick glacier.
How glaciers move: the flow of ice
Although ice appears brittle and rigid when handled, like many solids it is able to deform slowly over time by a process known as plastic flow or creep. Bonds within the ice crystals are able to disconnect and reconnect so that water molecules can move past each other. Over time, the ice crystals become aligned, giving the ice a fabric like a metamorphic rock.
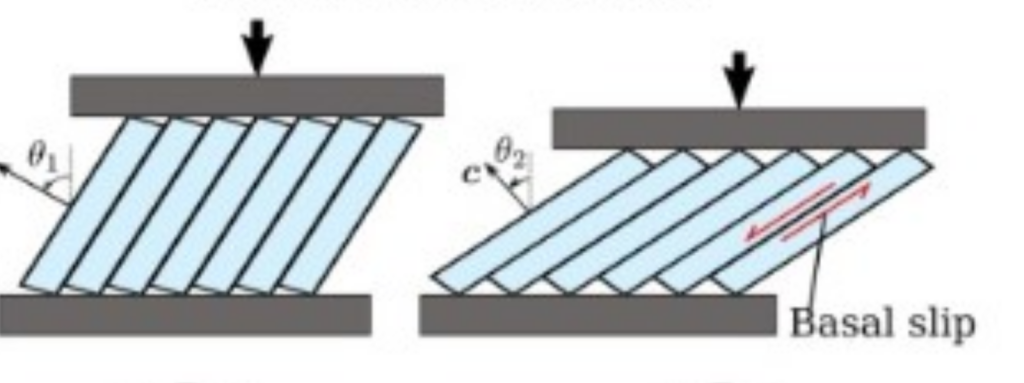
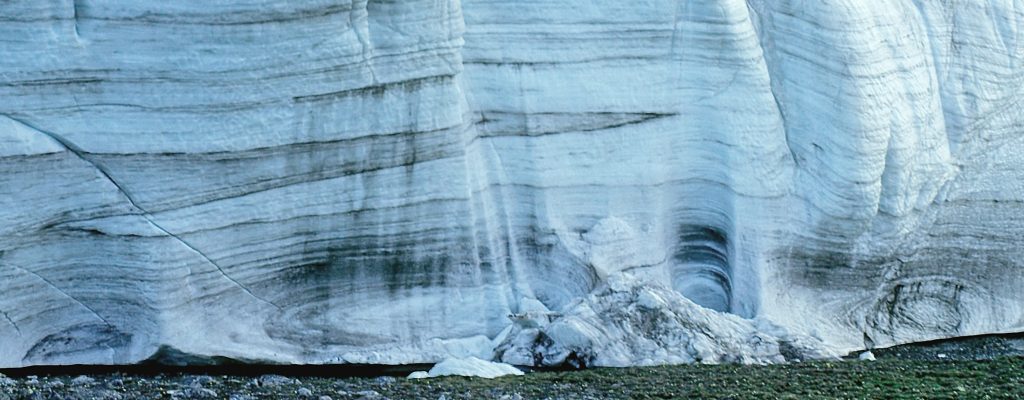
The intensity of deformation, or shearing, is greatest near the base of the glacier, but the speed of movement is greatest near the surface. Ice flow is always laminar: ice crystals move along smoothly curving lines unlike the swirling movements that characterise turbulent flow in rivers.
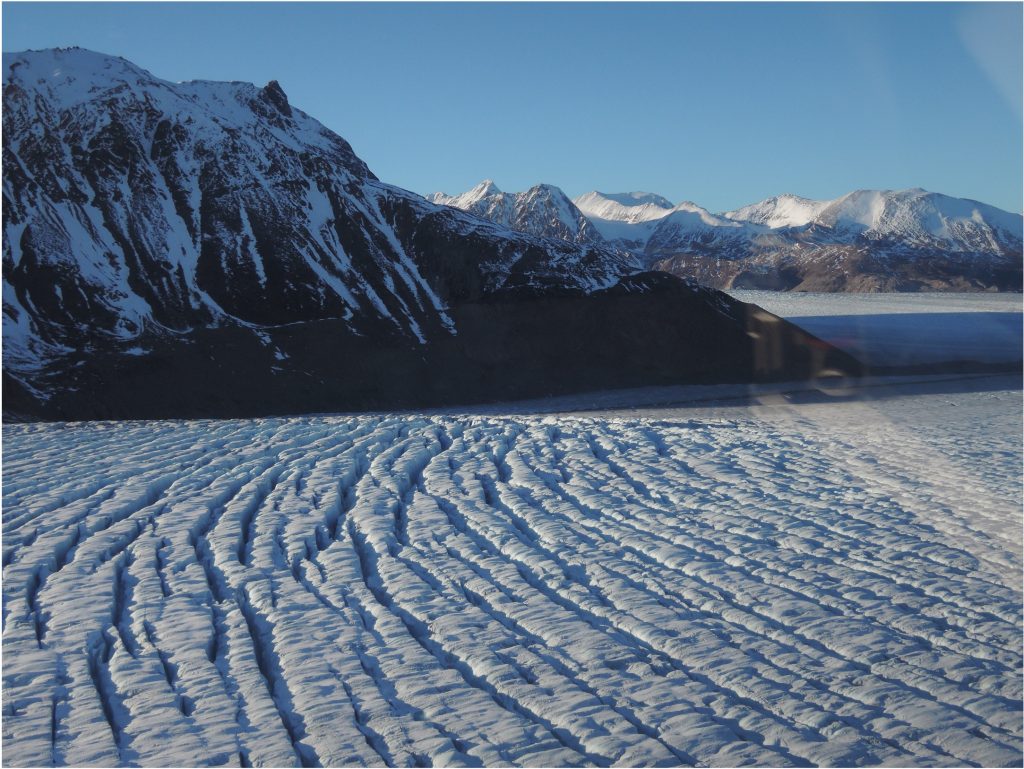
Low pressure encourages brittle fracture, rather than plastic flow, so most glaciers have a brittle upper layer, about 50 m thick. If this upper layer is forced to deform, for example as ice accelerates or flows over buried topography, crevasses – deep and hazardous cracks in the ice – may form.
The basal surfaces of glaciers, where they sit on bedrock or sediment, are also important for understanding glacier flow.
- Warm-based (“temperate”) glaciers are close to melting point at the base, so liquid water is present.
- Cold-based (“polar”) glaciers are below the melting point at their bases. No water is present, and the ice is frozen on to bedrock.
- Poly-thermal glaciers may have warm-based and cold-based areas.
In polar regions, glaciers are typically cold-based and frozen on to bedrock, but glaciers in temperate regions are typically warm-based; water present at the base enables them to slide. In addition, sometimes there is sediment — sand, gravel, or mud — at the base of a glacier, and this can deform as well, adding to the total rate of glacier movement.
The speed of the glacier is therefore determined by the combination:
Internal flow + basal sliding + deformation of underlying sediment.

The speed of the glacier is therefore determined by the combination:
Internal flow + basal sliding + deformation of underlying sediment.
Typical alpine valley glaciers move at speeds of a few tens to a few hundreds of metres per year. However, some surging glaciers have been observed to move at rates of 30 metres per day (more than 10 km per year).

Accumulation, ablation, and the snowline
The higher areas of a typical glacier are the site of net ice gain or accumulation: the addition of snow and its conversion during burial through névé and firn to glacier ice. In theaccumulation zone[2], the amount accumulated in the colder months exceeds the amount lost during the warmer months; If we track an individual ice crystal in this area, the accumulation zone, it moves downward within the glacier as it is buried and starts to move down slope.
The lower areas of a typical glacier are the site of net ice loss or ablation: loss of ice at the base, the top, and at the terminus. In the ablation zone[3], the amount of ice lost during warmer months exceeds the amount accumulated in colder months. If we track ice crystals in the ablation zone, they move outward towards the edges of the glacier, as ice is removed. The terminus, the line that marks the lower limit of the glacier, may advance or retreat over time as the glacier lengthens or shortens.
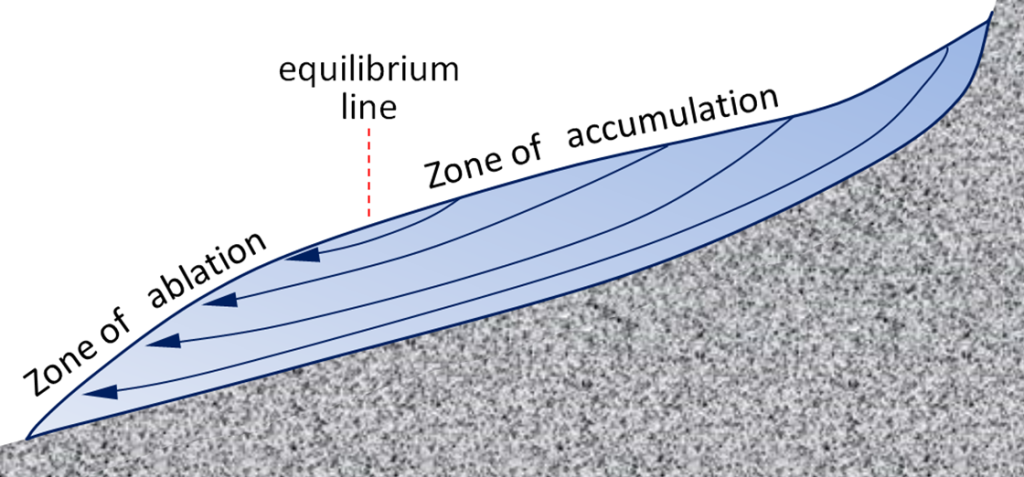
The zone of accumulation and the zone of ablation are separated by the equilibrium line, also known as the snowline. The equilibrium line is where accumulation and ablation, when averaged over a few years, are equal. At the end of summer melting, the snowline can typically be recognized as a boundary between white snow cover that persists in the accumulation zon, and darker solid, sometimes sediment-laden ice that is exposed in the ablation zone.
The equilibrium line, there is neither net accumulation nor net ablation of ice, so the flow of ice is predicted to be exactly parallel to the ice surface.
Mass balance, advance and retreat
Ice in glaciers are always moving downslope, but glaciers are said to advance (grow) or retreat (shrink) depending on the relationship between:
Accumulation: Addition of mass to a glacier through:
- Snow, hail, freezing rain
- Avalanching of snow from adjacent slopes
Ablation: Removal of mass from a glacier through:
- Melting
- Sublimation (direct evaporation of ice into water vapour)
- Iceberg calving
- Wind erosion
Mass Balance is the difference between the amount of material that a glacier accumulates and the amount lost by ablation. If there is more accumulation than ablation, the equilibrium line moves down. If ablation exceeds accumulation, the equilibrium line moves up. In summary:
- Advance: Gains exceed losses (accumulation > ablation): positive mass balance (glacier thickens and advances, equilibrium line moves down)
- Retreat: Losses exceed gains (accumulation < ablation): negative mass balance (glacier thins and retreats, equilibrium line moves up)
It is important to realize that even when an ice sheet retreats, ice itself does not move back up hill. Ice continues to flow downhill, but ablation at the terminus exceeds the rate of supply of ice from above, and the terminus itself retreats. Most (though not all) glaciers in the 21st century are in a state of retreat; recent climate change has led to decreased accumulation and increased ablation. There are exceptions. In some areas, recent warming of the atmosphere has led to increased humidity and increased snowfall, causing accumulation to increase fast enough to offset losses through ablation.
<Mass balance diagram for glaciers around the arctic from US Global climate research program>
Rapid glacier retreat has global consequences, because glaciers trap large volumes of water above sea level. When this water is returned to the oceans, sea level rises. Sea level rose ~1.7 mm/yr prior to 1993; in the early 2020s this rate has increased to 3.3 mm/yr. About 60% of the rise is directly due to melting glaciers. If all the remaining land-based glaciers were to melt, sea level would rise by 60–70 m. (Note that floating ice does not have a direct effect on sea-level when it melts, because floating ice displaces its own weight of water. The meltwater produced by floating ice fits into the space left when the ice melts.)
Types of glaciers
Glaciers are generally classified into two large categories based on whether or not they are confined between mountains. Alpine glaciers are controlled by topography such as valleys and mountain peaks whereas continental glaciers are so large that the surface of the glacier appears to be mainly unaffected by topography.
Continental glaciers
Ice sheets
Ice sheets are huge masses of glacial ice where flow is unconstrained by topography. At the present day, Earth’s only ice sheets are in Greenland and Antarctica.

Ice sheets have their highest elevation roughly at the centre, and flow is from this highest elevation area radially outwards.
Ice sheets are generally slow moving (millimetres per day, or metres to tens of metres per year. However, if an ice sheet is ‘dammed in’ by mountains, ice may escape between the mountains through outlet glaciers that resemble alpine valley glaciers. This occurs particularly around the Greenland ice sheet, where some outlet glaciers are locations of faster movement, locally up to 30 m/day.
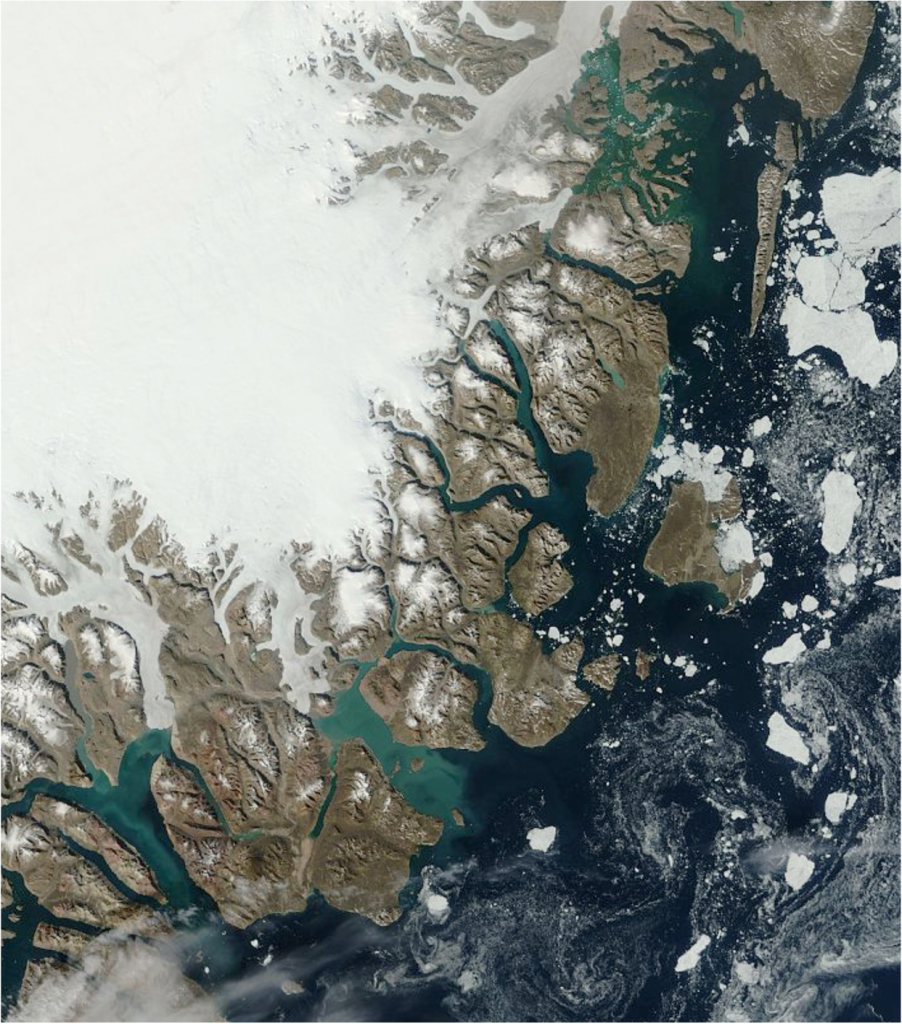
Antarctica has 90% of Earth’s ice, contained in two ice sheets: The larger of the two is the East Antarctica Ice Sheet, which contains most (over 90%) of the ice in Antarctica (and therefore over 80% of Earth’s ice). It is separated by the Transantarctic Mountains from the West Antarctic Ice sheet, which is much smaller, but which has been studied in more detail. The Transantarctic Mountains locally poke out of the ice surface (as nunataks — mountain peaks surrounded by glacier ice) along the join between the East and West Antarctic ice sheets. A small additional component, sometimes regarded as an ice sheet in its own right, extends northward from West Antarctica towards South America on the Antarctic Peninsula. Because of its small size and northern position, ice in the Antarctic Peninsula is particularly affected by climate change.
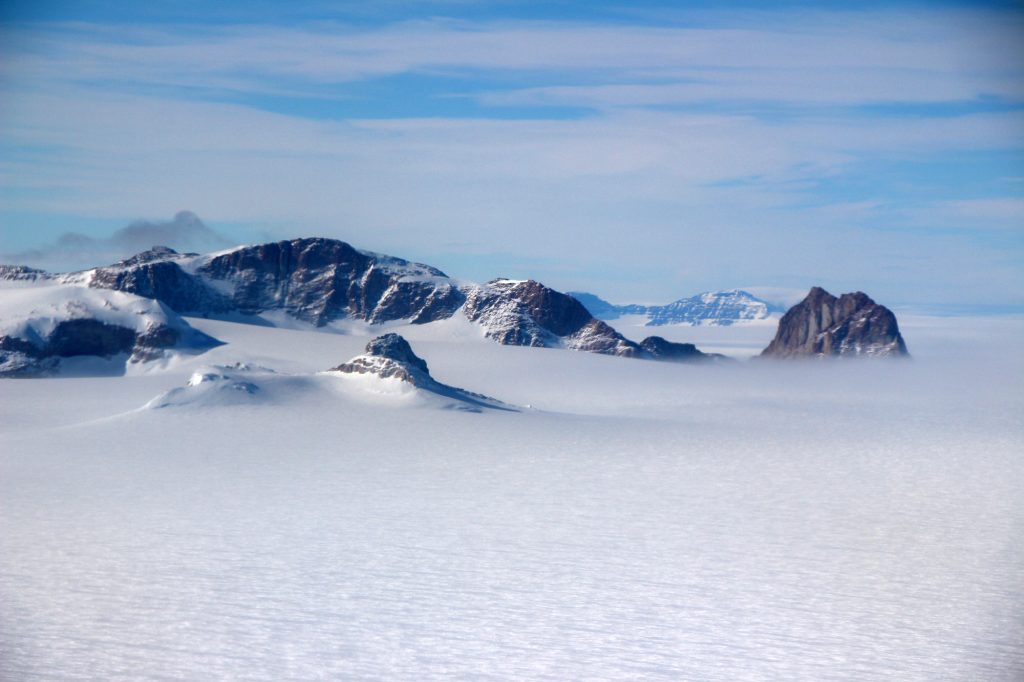
Measurements of the composition of marine sediments suggest that that the Antarctic Ice Sheet started to form at about 35 Ma. At the present day, ice in the Antarctic Ice Sheet is up to 4.8 km thick, based on measurements using ice-penetrating radar, but the average thickness is a little over 2 km. Beneath the thickest parts of the ice, geothermal energy from below, combined with elevated pressure, creates conditions for melting, producing subglacial lakes, that have been sampled by drilling. Beneath the thickest parts, particularly of the West Antarctic Ice Sheet, the base of the ice is well below sea level, but this is partly due to the weight of the ice. If the ice were to melt, the water returned to the oceans would cause a worldwide sea-level rise of about 58 m, but the lithosphere below Antarctica would undergo isostatic rebound so that Antarctica would lie mostly above sea level. The two Antarctic ice sheets would contribute differently to these effects. The West Antarctic Ice Sheet would contribute only about 5 m of global sea-level rise, but much of its bed is currently below sea level. Because of this is far more susceptible to collapse than the much larger East Antarctic Ice Sheet, which is largely based above sea level. However, if the East Antarctic Ice Sheet were to collapse, it would raise sea level globally by ~53 m.
Parts of the Antarctic Ice Sheet extend into the neighbouring seas as ice shelves. Because ice is less dense than water, it floats as it moves offshore; the line where the ice begins to float is called the grounding line. Because of the lack of resistance to flow, the ice spreads out and the upper surfaces of the ice shelves are much flatter than grounded glaciers. Ice shelves lose ice mainly by calving: large pieces of ice break off to become icebergs. Ice shelves are particularly vulnerable to climate change, as they are warmed both by the air above and by the water below. It is believed that ice shelves help to hold back the flow of ice in the grounded glaciers behind them, so that when Antarctic ice shelves are lost, this results in faster flow of other portions of the ice[4].

Ice shelves should not be confused with sea ice. Ice shelves are floating ice which has flowed from land-based glaciers, ultimately derived from snow, whereas sea ice is ocean water that has directly frozen on the sea surface.
Because ice shelves and sea ice float on water, their melting does not cause isostatic rebound, and does not directly impact sea level, unlike the melting of grounded ice. This is because the ice contracts as it melts, fitting into the same space as the submerged parts of the floating ice[5].
<image of Greenland ice sheet preferably with flow velocity shading>
The Greenland Ice Sheet is the Earth’s only other ice sheet at the present day (though others existed in the past). It’s much smaller than the Antarctic Ice Sheet, having enough ice to raise sea level by an additional 7.4 m if it were to completely melt. It does not have broad floating ice shelves at its margins, as the ice margin is mainly on land. However, the Greenland Ice Sheet has a large number of outlet glaciers, some of which reach the sea and produce icebergs. These outlet glaciers include some of the fastest moving ice on Earth — up to 30 m per day. The Greenland Ice Sheet is currently losing ice at an accelerating rate, much faster than Antarctica, and it is the single largest contributor to rising sea level worldwide.
<Greenland outlet glacier>
Ice caps
Ice caps are essentially miniature ice sheets: gently dome-shaped masses of ice with area less than 50,000 km2. This corresponds to a diameter less than about 250 km. Like ice sheets their shape is not controlled by the topography of surrounding mountains, although some sit on top of mountainous areas. Well known examples of ice caps occur in Iceland and in the Canadian Arctic.
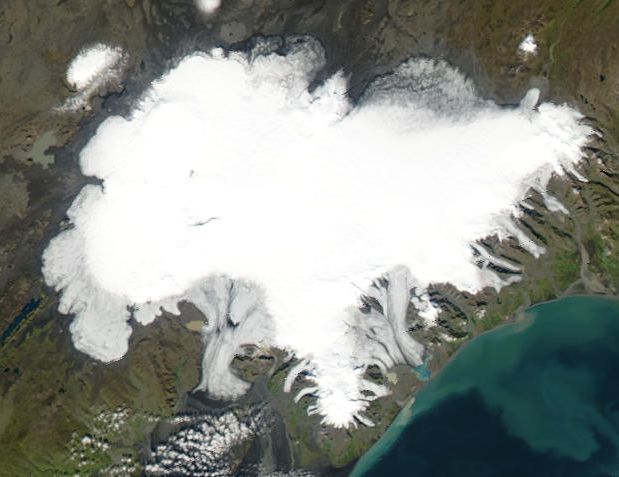
Alpine glaciers
Ice fields
Ice fields are areas of very high ground in mountain regions may be the source of several valley glaciers that flow outwards in different directions from a mass of glacier ice. Ice fields are somwhat similar to ice caps, but are usually smaller and are more constrained by topographic features such as mountain peaks, whereas ice caps have a smooth dome shape that overtops mountain peaks. Columbia Icefield is the largest of ~30 named ice fields in the Canadian Rockies.
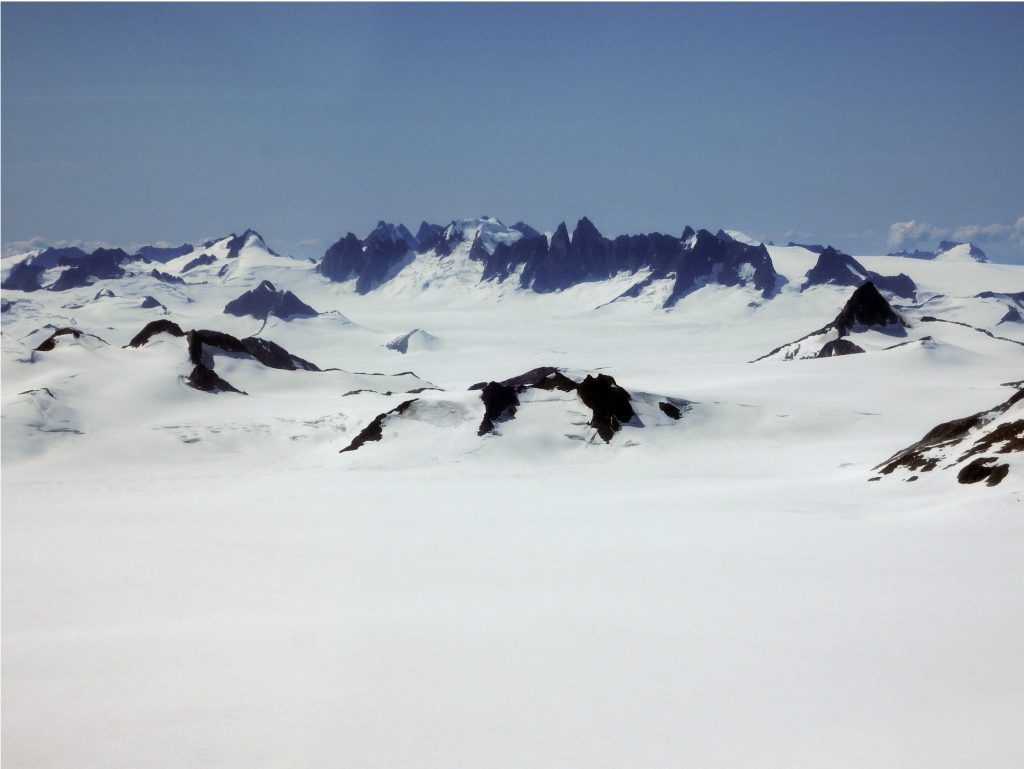
<https://earthobservatory.nasa.gov/images/90341/north-patagonian-icefield>
Cirque, valley, and fjord glaciers
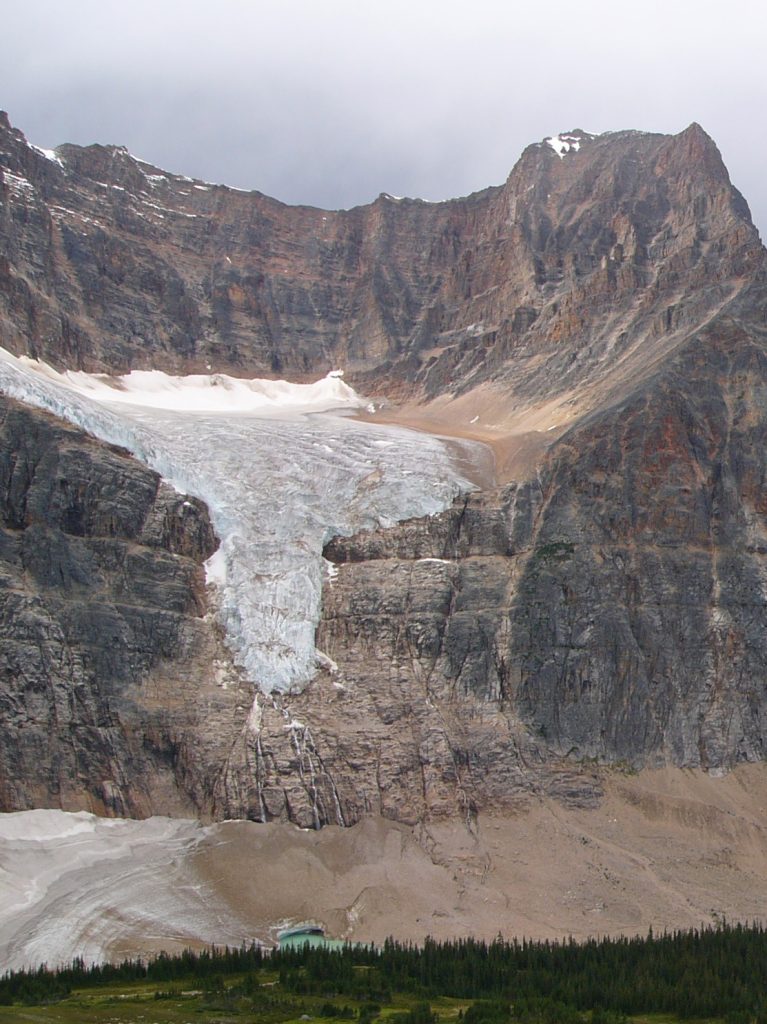
Cirque glaciers occupy bowl-shaped depressions (cirques) on the sides of mountains that are themselves formed by glacial erosion. They are often approximately equant (i.e. length and width are roughly equal).
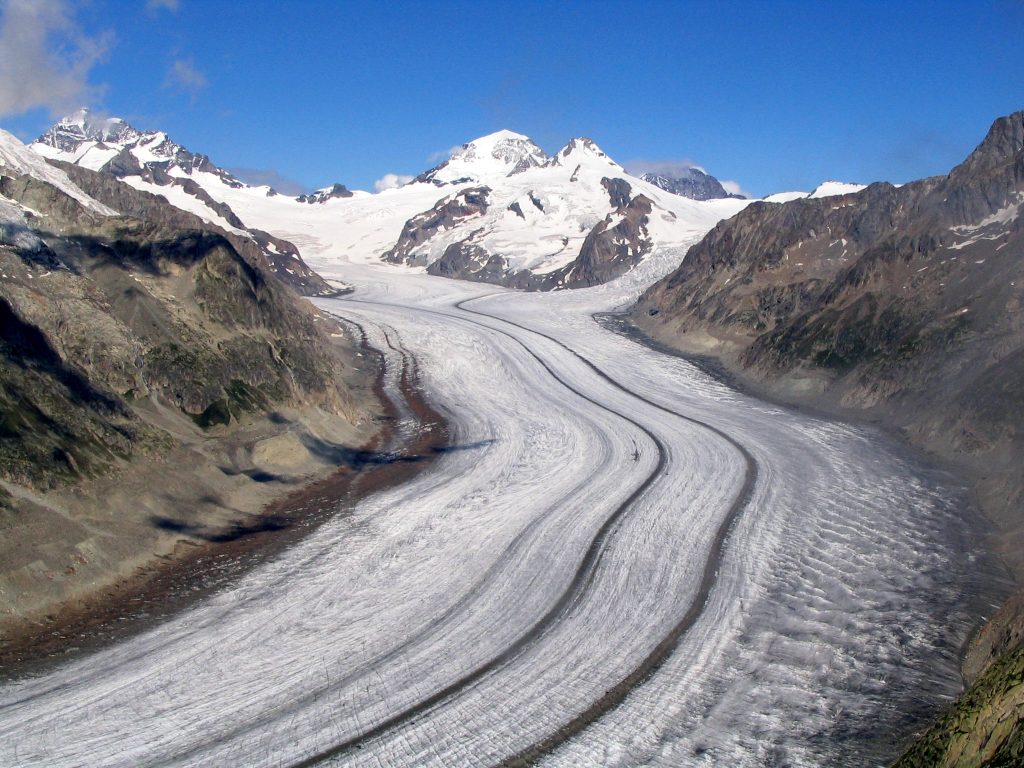
Valley glaciers are often regarded as the most typical type of Alpine glacier. They form when ice that accumulated in topographically high regions flows down mountain valleys toward lower ground. They are typically much longer (measured in the ice-flow direction) than they are wide. Below the equilibrium line, valley glaciers that have formed by the convergence of one or more distributary glaciers typically show a pattern of stripes on their surfaces (medial moraines) due to the presence of sediment, illustrating the characteristic laminar flow of glaciers.
Fjord or tidewater glaciers are similar to valley glaciers but flow into water, usually seawater. These glaciers may undergo ablation by calving to produce icebergs.
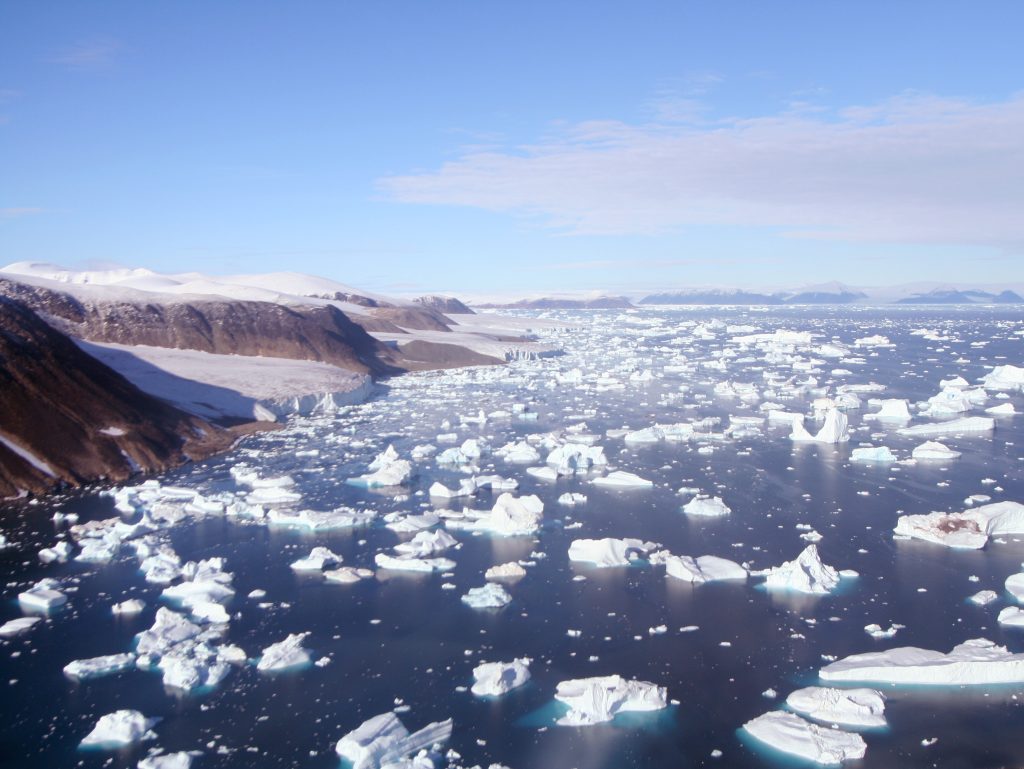

Piedmont glaciers
When one or more valley glaciers spread out at the downstream end into a broad lowland they may form a less confined, spreading glacier known as a piedmont glacier. Piedmont glaciers are possible analogues for the large, warm-based ice sheets that covered much larger areas of North America and Europe during the last glacial maximum around 20,000 years ago.

Weathering, erosion and deposition by glaciers
In addition to their interactions with the atmosphere and the rest of the Hydrosphere, glaciers have important interactions with the solid Earth — the Geosphere — because they carry out weathering, erosion and deposition of sediment.
Weathering and erosion processes
Glaciers predominantly carry out physical weathering processes. Cold temperatures generally slow down chemical reactions, so chemical weathering processes are generally less important in glacial environments. Several processes contribute to physical weathering by glaciers.
Freeze-thaw cycles
Warm-based glaciers are powerful agents of physical weathering, particularly because a small rise in either pressure or temperature during ice flow can produce melting. The resulting melt-water penetrates pore spaces in underlying rock, including spaces between the grains of porous rocks, and cracks in less porous rock, where it may freeze. The resulting expansion, known as frost wedging, forces the rock apart, creating fragments of multiple sizes.
Plucking
Once the water surrounding a fragment has frozen, the fragment is immersed in ice that is relatively strong. As the ice flows onward, such fragments are pulled away from bedrock, a process known as glacialplucking. Plucking is particularly effective on the downstream sides of bedrock hills that stick up into the ice, where it tends to produce irregular, somewhat jagged surfaces as angular fragments are pulled away.
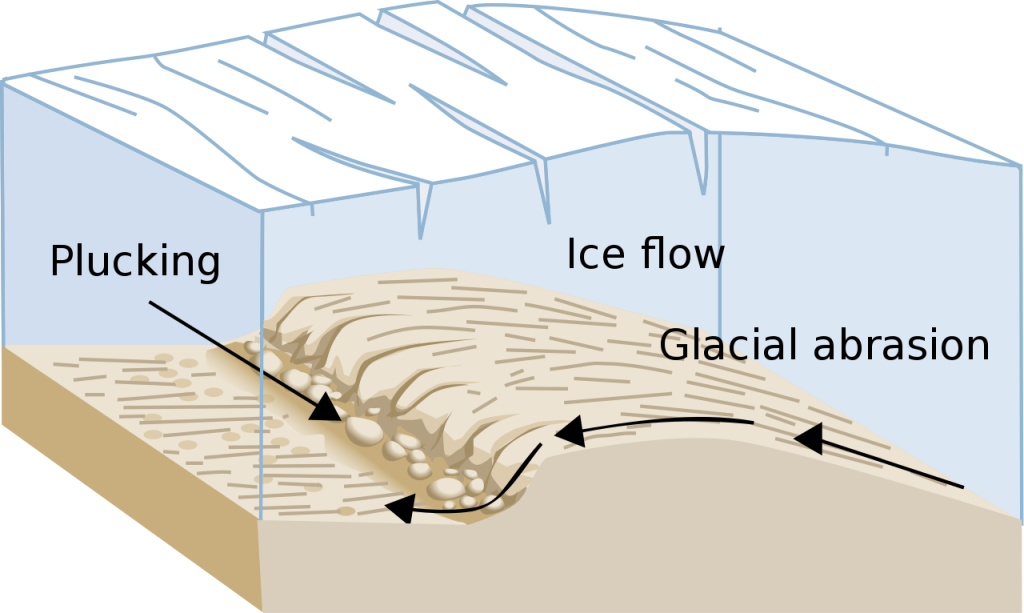
Abrasion
Fragments of rock that have been picked up by ice by freeze-thaw and plucking typically continue to travel close to the bottom of the glacier, where they may be dragged along the bedrock surface, grinding away even more rock in the process known as abrasion.

Abrasion is particularly effective on the upstream sides of hills that stick up into the ice.
Erosional features
The processes listed above combine to make warm-based glaciers potent agents of erosion. Landscapes that were recently covered by glaciers typically show a variety of erosional features, ranging from scratches and grooves only millimetres across to characteristic styles of valley that may be kilometres wide. (Much less erosion occurs beneath cold-based glaciers, because the ice is effectively frozen to bedrock and the basal layer of ice barely moves.)
Striations or striae
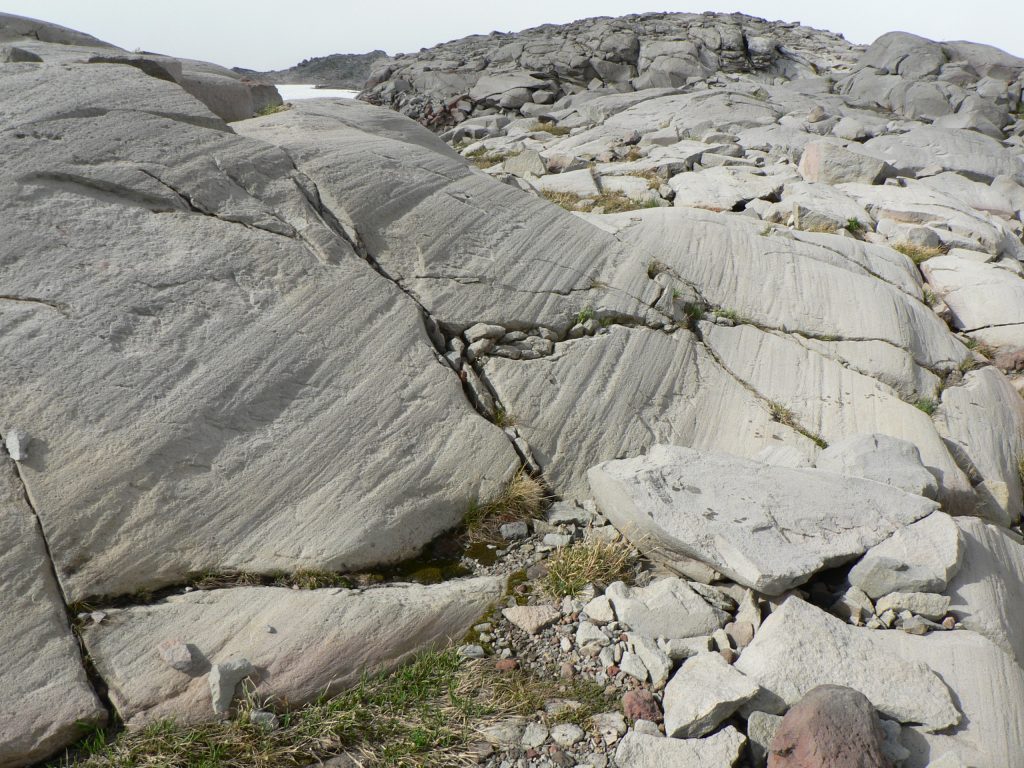
Abrasion by large chunks of rock typically makes grooves in bedrock surfaces that have been covered by glaciers. These grooves are called striae (plurial of a Latin word stria for a groove) or striations. Striations may be accompanied by transverse notches called chatter marks, formed where fragments have dug in to or fractured the rock surface. Chatter marks typically have a steep side that occurs at the downstream edge of the notch, facing upstream. The combination of striations and chatter marks may be used to make maps that show the former ice-flow direction in areas that were previously covered by glaciers.
Roches moutonnées
As a result of the combination of abrasion on the upstream side and plucking on the downstream side, bedrock hills and other upstanding rocks that have been affected by glacial weathering and erosion often show a characteristic shape, with a smooth, arching upstream end and an irregular downstream end. Such a hill is called a roche moutonnée (from a term in French, literally meaning something like “rock made into a sheep fleece”)
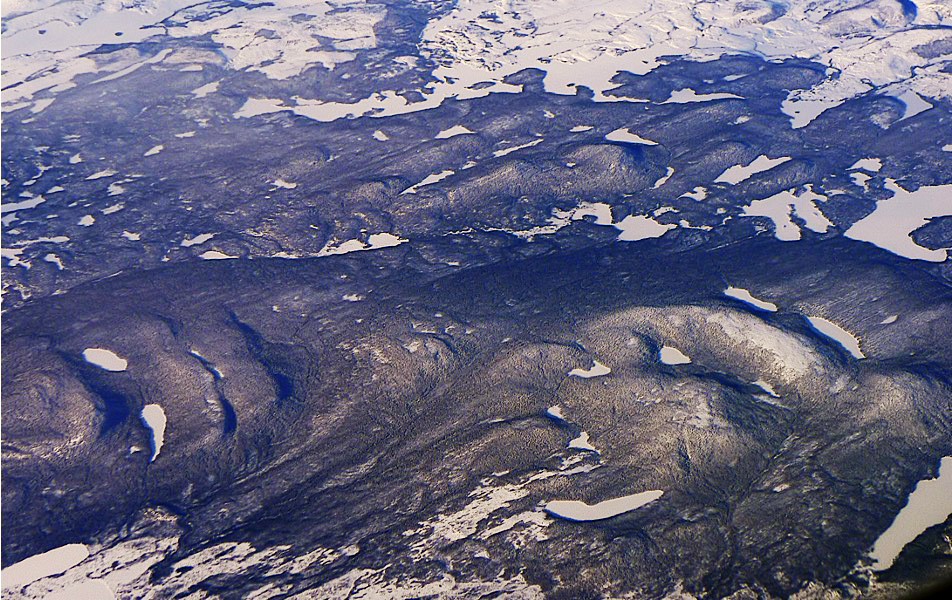
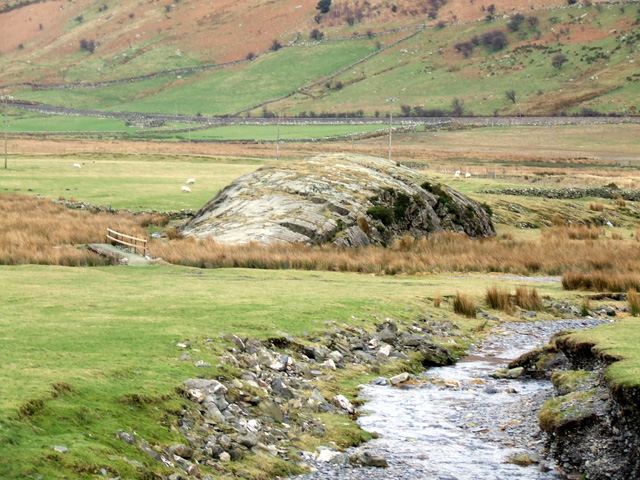
Glacial valleys
Glaciers tend to fill their valleys much more deeply than rivers, with the result that valleys that have been carved by glaciers typically have a distinctive U-shape in cross-section. This contrasts with the typical V-shaped profile of a river valley, where slope processes (mass wasting) dominate erosion on the valley sides.
Where glaciers join, the top surfaces of the two glaciers tend to be approximately level. If one glacier is much shallower than the other, there will be a distinct step in the glacier bed where the small glacier joins the larger one. Once the ice has melted, this results in a hanging valley at the top of a steep slope on the main valley side.
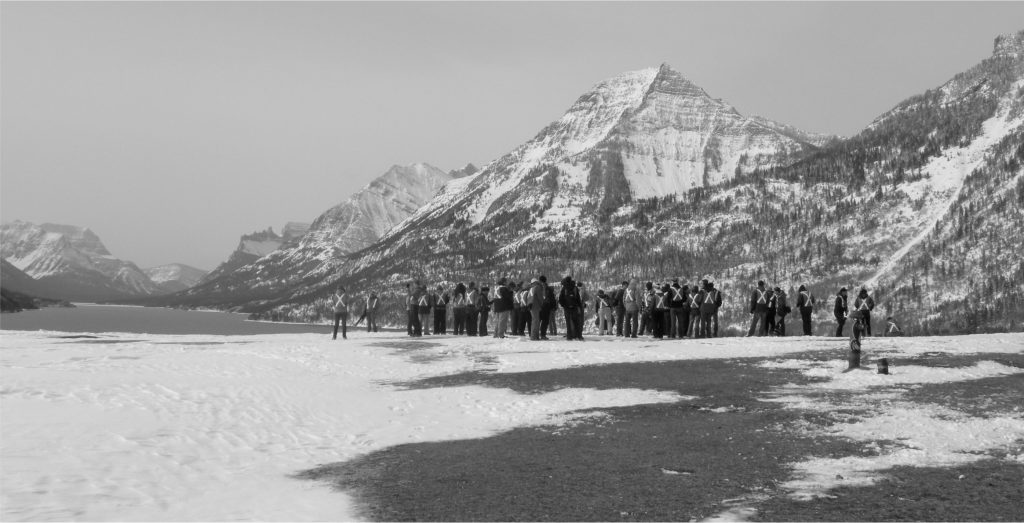
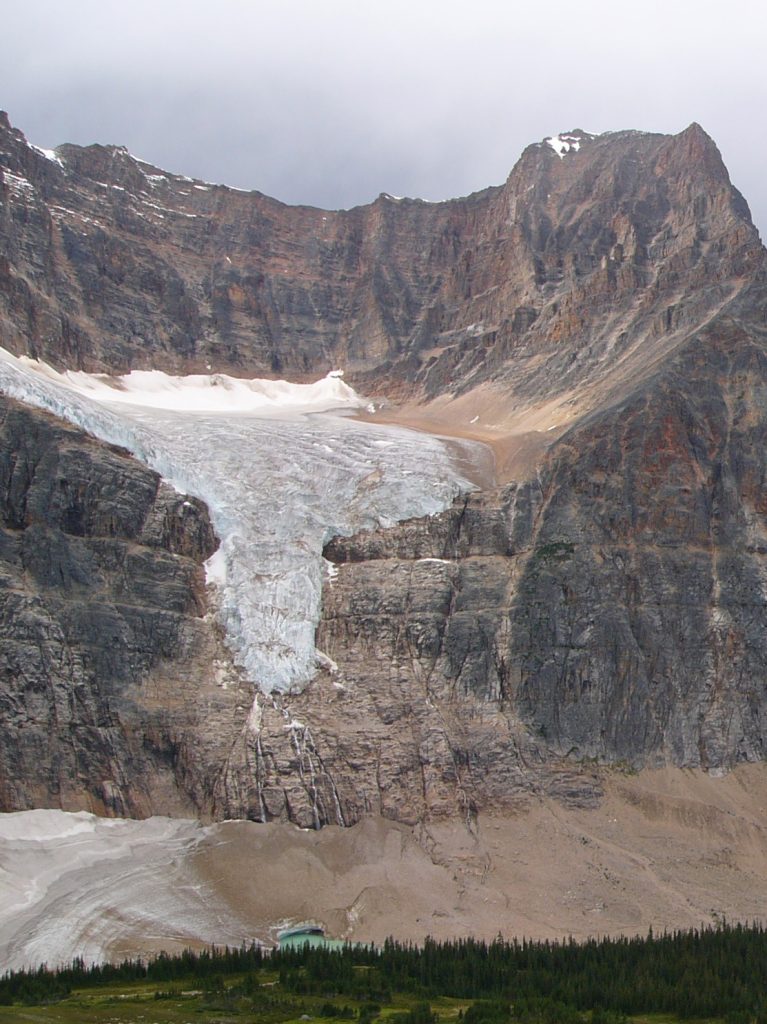
Cirques, arêtes and horns
Cirques are rounded, bowl-shaped valleys formed by glacial erosion at a glacier head. Cirques that feed into a larger valley glacier commonly form hanging valleys.
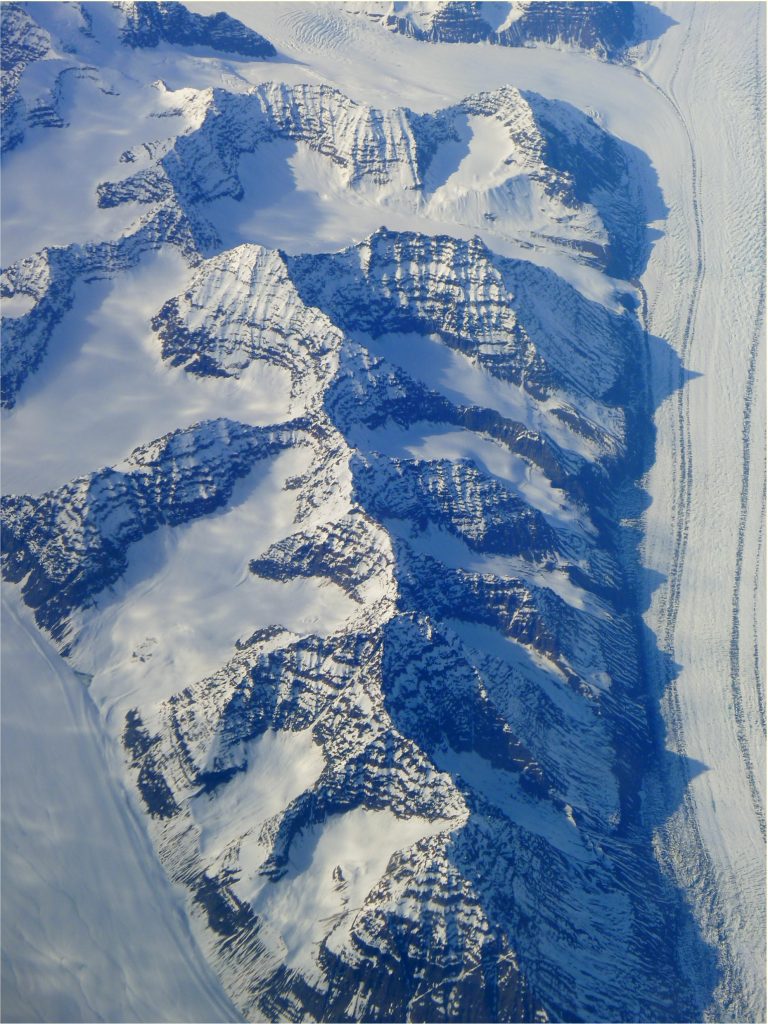
The ridges that form between glacial valleys are commonly made of frost-shattered rock and may be particularly steep and jagged; they are known as arêtes. Peaks where three or more arêtes meet are known as horns. The Matterhorn in the Alps is a typical example.
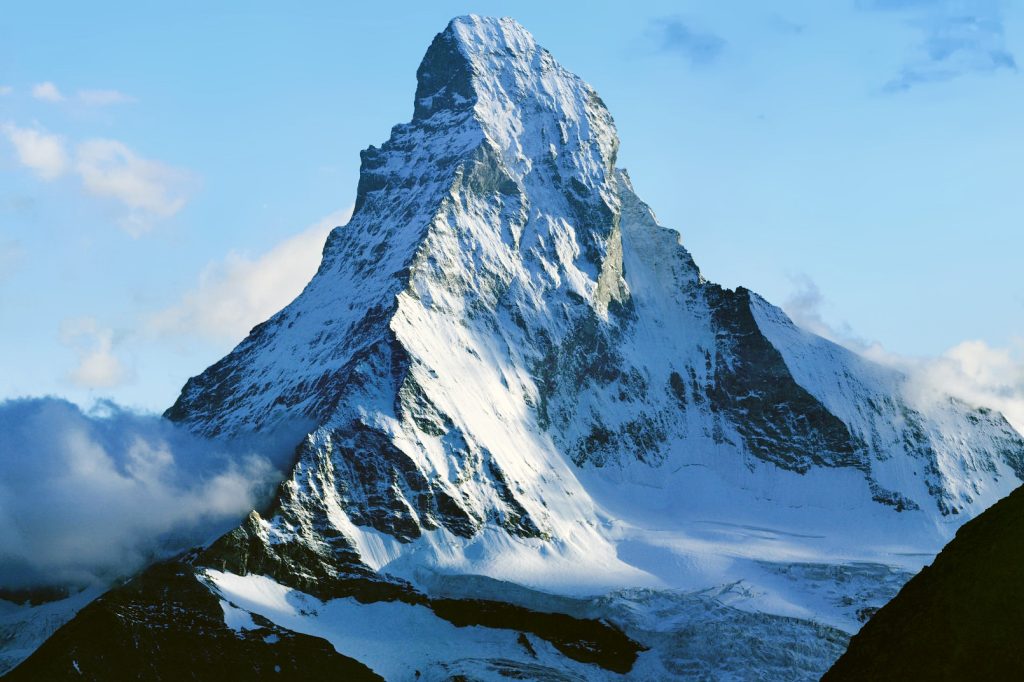
Outburst flood channels
Meltwater lakes (see below) are common around the edges of glaciers. Some former glacial lakes were extremely large. The Laurentide Ice Sheet, which covered much of Canada during the last glacial maximum, was surrounded by a number of ice-marginal lakes, trapped behind glacier ice. Melting led to catastrophic outbursts of water that carved deep channels in the landscape, known as outburst channels.
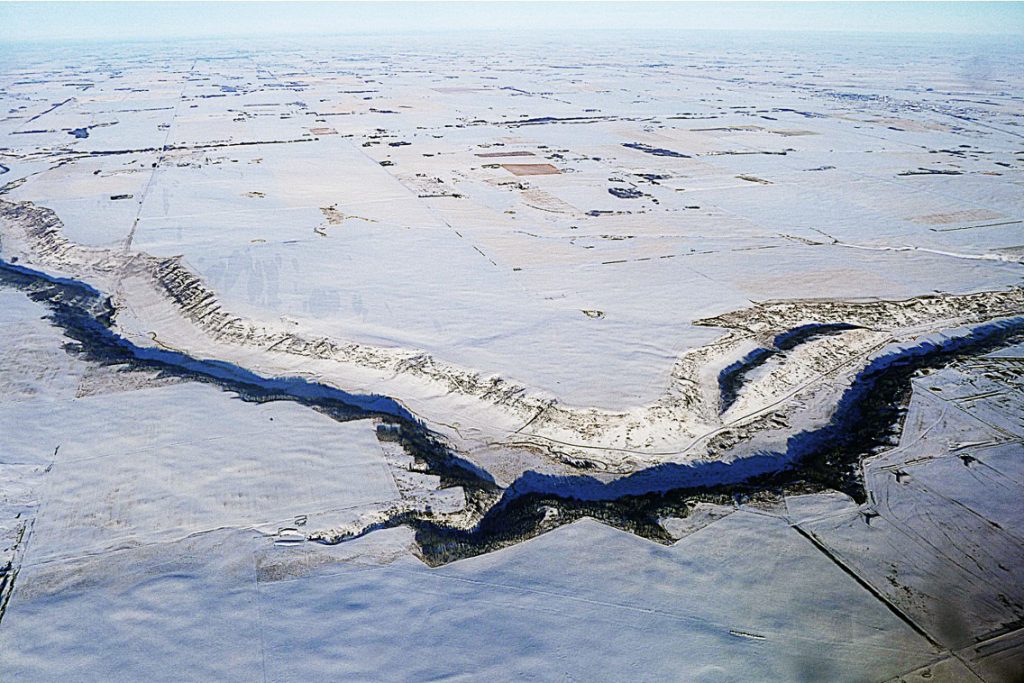
Direct deposition by ice: till and till landforms
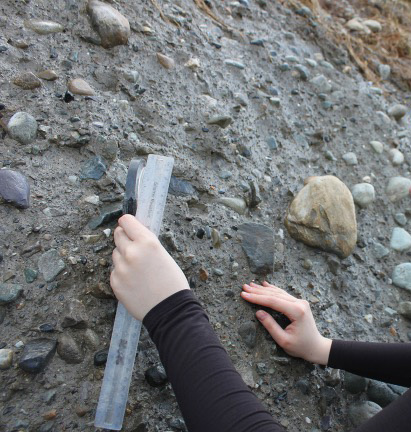
Till
Because ice is strong enough to carry rock fragments of almost any size, and because glacier flow is laminar, glaciers do not sort the sediment they carry: grain-sizes from the finest mud to huge boulders may be carried together. Therefore, sediment deposited directly from ice, known as till, is recognizable from its lack of sorting. (An old British name for till is boulder clay, a name which captures the wide range of grain sizes.) Till typically also lacks bedding, though indistinct layers may be present if there are changes in the sediment content of the ice as till is deposited.
Moraines and drumlins
Till is concentrated in parts of the landscape where ice melts. After the retreat of glaciers, the resulting landforms may give clues about the former flow of ice. The term moraine is used to describe many different accumulations of till.
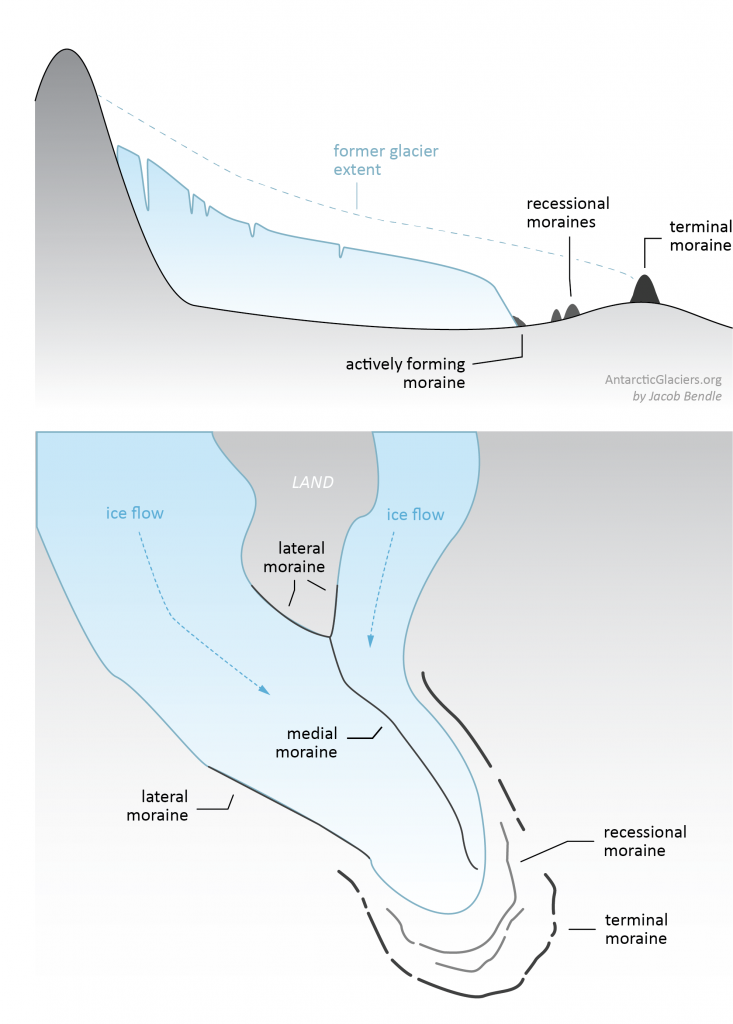
- Lateral moraines are bands of till that accumulate on either side of a valley glacier. Much of the material is actually supplied by mass wasting: rock falls from the steep valley sides and accumulates on the top surface of the glacier as a band on each side.
- Medial moraines form when two valley glaciers merge. The former lateral moraines now form a band of sediment-laden ice down the middle of the merged glacier. A large valley glaciers that formed from the merger of many tributary glaciers may have many medial moraines of different sizes, giving the glacier a characteristic striped appearance that illustrates the laminar flow of the ice.
- End moraines form at the terminus of the glacier, where ablation is typically fastest. Two varieties are named:
- The terminal moraine marks the maximum extent of a glacier;
- Recessional moraines are found up-slope from the terminal moraine after a glacier has retreated. They typically mark pauses in the retreat process, or periods of rapid till supply.
-
Lateral moraines and a proglacial lake in the Himalaya. By Bijaya2043 – Own work, CC BY-SA 3.0, https://commons.wikimedia.org/w/index.php?curid=33108487
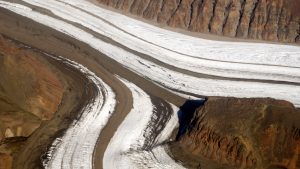
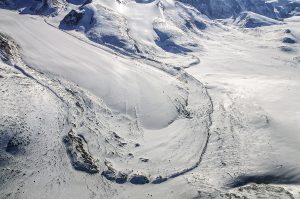
Drumlins are oval hills of glacial till, typically about a kilometre long, that cover landscapes formerly covered by ice. They are subglacial landforms, formed under warm-based ice sheets, as subglacial till was moulded by the overlying ice flow. They are typically slightly steeper on the upstream side, and slope more gently in the downstream direction of ice flow.[6] The origin of drumlins is controversial. Their formation is hidden from view beneath ice, and because the two remaining modern ice sheets (Greenland and Antarctica) are mostly cold-based, there is a lack of good modern analogues. There are two main hypotheses (with many variations). Either drumlins are constructed by the flow of ice which moulds an underlying blanket of till by a poorly understood process, or they are erosional remnants of a once-continous thicker sheet of till, left behind when fast-flowing water or surging ice streams removed till from a network of channels.
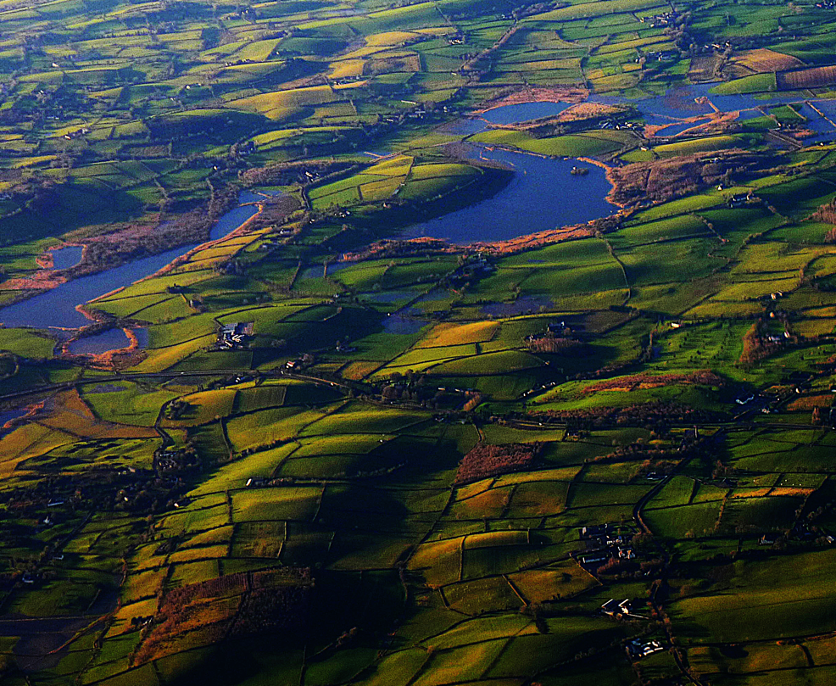
<to add: LiDAR imagery of Drumlins NS & elsewhere>
Erratics
Sometimes ice may deposit huge, isolated blocks of rock that are much larger than any surrounding till deposits. Typically, such blocks are of rocks that are completely foreign to the surrounding bedrock, in which case they are known as erratics. If the rock-type of an erratic is distinctive, and can be traced back to a source area, it may provide clues to the long-distance routes followed by ice in ancient glaciers
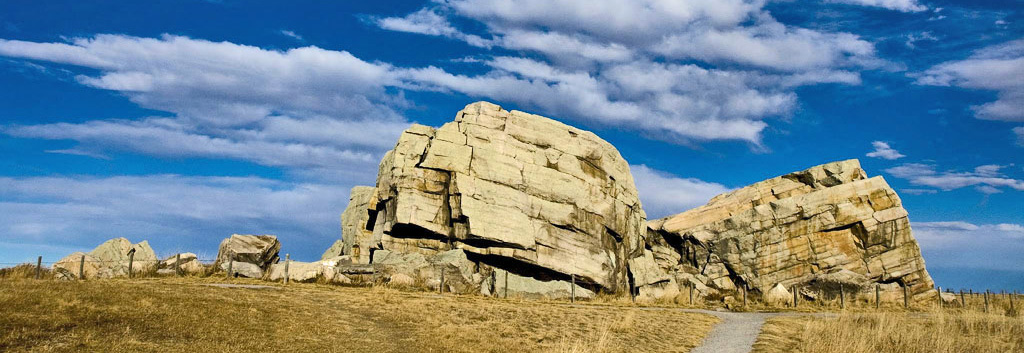
Deposition by meltwater: glaciofluvial and glaciolacustrine deposits
Large amounts of water are produced during glacier ablation, and this water transports sediment on the glacier surface, at the glacier bed, and beyond the terminus of glacier ice. Sediment deposited by flowing water can be distinguished from till because water-deposited sediment is typically better sorted, and shows bedding, lamination, and other sedimentary structures similar to those formed by rivers and lakes. Sediments formed by water flowing from glaciers is described as glaciofluvial, whereas sediment deposited in lakes developed around glaciers is glaciolacustrine. In many landscapes that were formerly glaciated, and are now occupied by humans, glaciofluvial sediments are sought after, because their high porosity makes them good aquifers, and also because they provide aggregate that is much more suitable for construction uses than poorly sorted till.
Eskers and kames
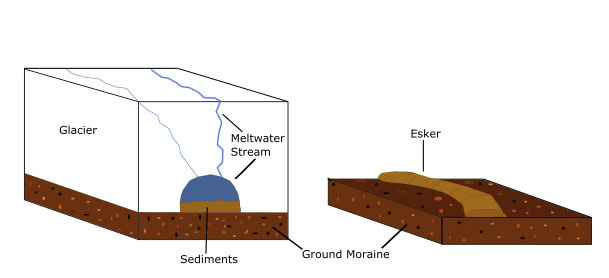
Ablation begins well above the terminus of most glaciers, so water is present both on top of and below glacier ice. Water below the ice (in the subglacial environment) may flow through tunnels at or near the base of a glacier, transporting both bed load and suspended load. Bed load may be deposited on the floor of the tunnel; after glacier retreat, this sediment may remain on the landscape, not filling a channel like normal deposits, but forming an upstanding ridge called an esker.
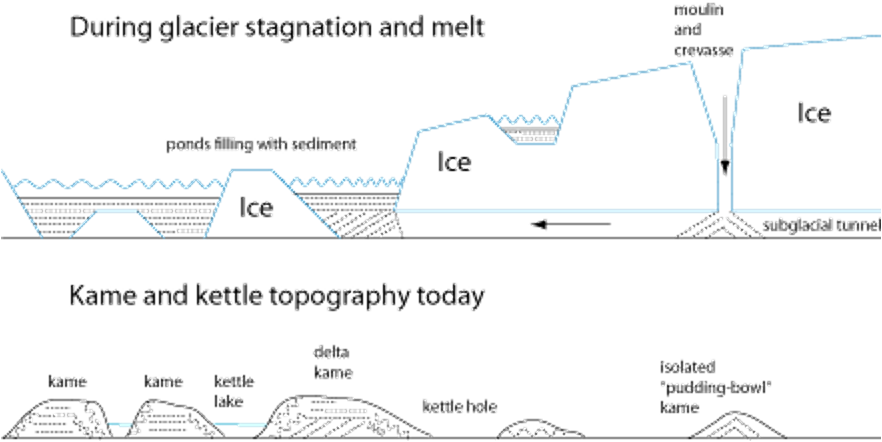
Water is also present on the top of glacier ice, both as streams and as ponds and lakes. Well sorted sediment may be deposited in these features too. After glacier retreat the sediment is left in the landscape as hills of well-sorted sediment in apparently random locations, often surrounded by till from the base of the glacier. These features are known as kames. Kames may be roughly equant but elongated kame terraces, formed by streams that flowed along the edges of valley glaciers, are also common. Kame terraces may be difficult to distinguish from eskers, but because ice has been ablated from beneath kames, the sediment layers in kames are typically somewhat more disturbed than those in eskers.
Outwash plains
Large amounts of sediment are deposited by flowing water beyond the terminus of a glacier. Braided streams are much more common in this environment than meandering streams, probably because the lack of vegetation and the strongly seasonal flow of water and sediment combine to inhibit the formation of stable floodplains.
<view of outwash plain>
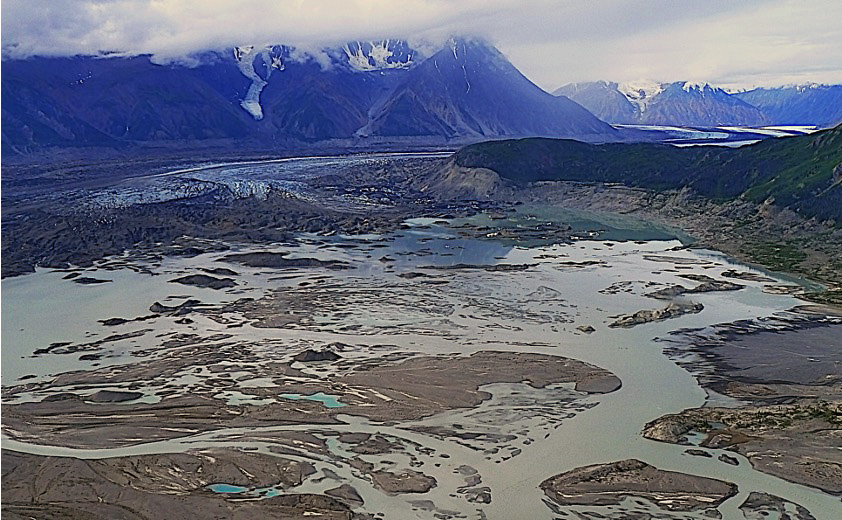
Glaciolacustrine and glaciomarine environments
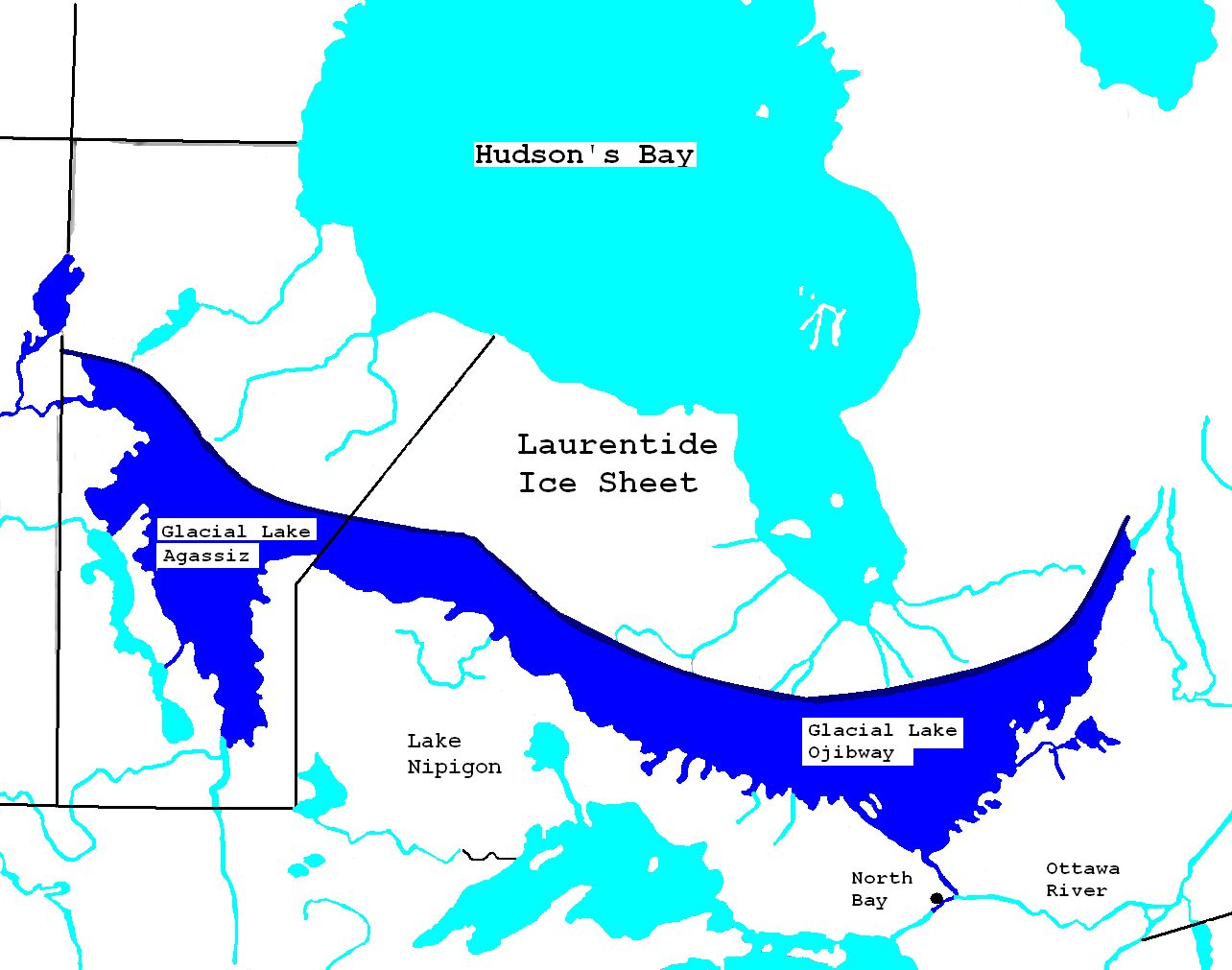
Lake that forms in front of or along the margins of a glacier are described as proglacial. They range in size from ponds only a few metres in diameter to huge lakes hundreds of kilometres across. Small proglacial lakes, typically formed where blocks of ice have been stranded in an outwash plain, are known as kettles. Much larger proglacial lakes, hundreds of kilometres across, occurred around the margins of the Laurentide Ice Sheet that occupied much of Canada during the last glacial maximum at about 20 ka. Water pooled in low areas at the ice margin because the lithosphere was depresed isostatically by the weight of the ice.
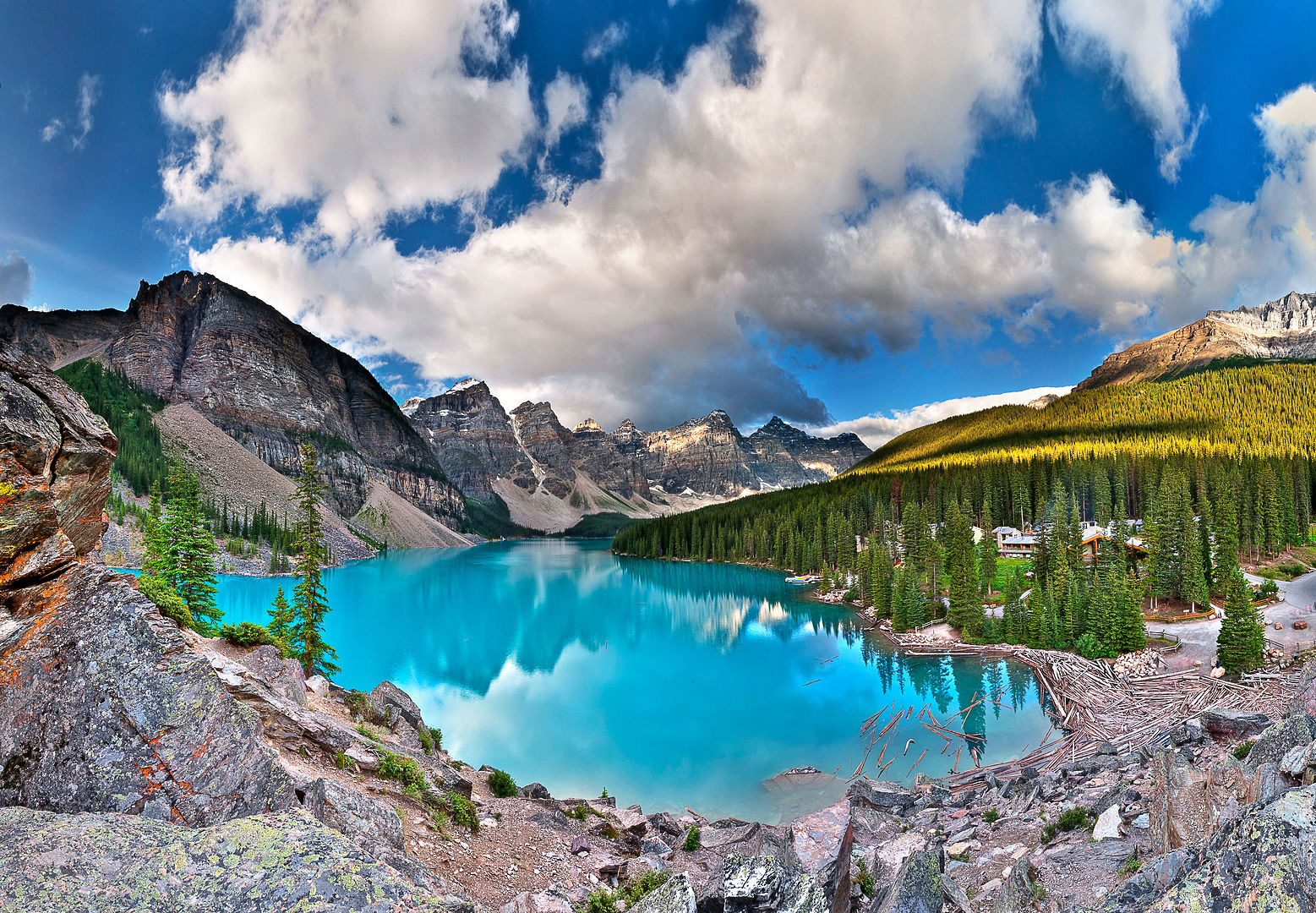
The finer-grained material produced by glaciers (known as rock flour) is suspended in such lakes and may give them distinctive colours. Chemical weathering is slow in glacial environments; therefore, when ompared with muddy sediment formed in warmer environment, proglacial rock flour typically contains a smaller proportion of chemically produced clay minerals and a larger proportion of fine-grained minerals preserved from the original source rocks.
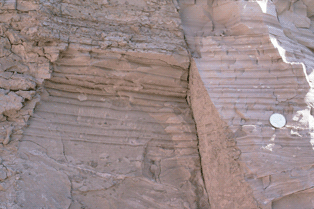
The supply of sediment to glacial lakes is strongly seasonal: large amounts of rock flour are delivered during summer melting; much less arrives in winter. As a result, the sediments deposited in such lakes may show fine laminae representing single years. Such laminae are known as varves. In some ancient glaciolacustrine deposits, the precise age of individual laminae can be determined by counting varves back through time from the present, one of the most precise methods of measuring geologic time.
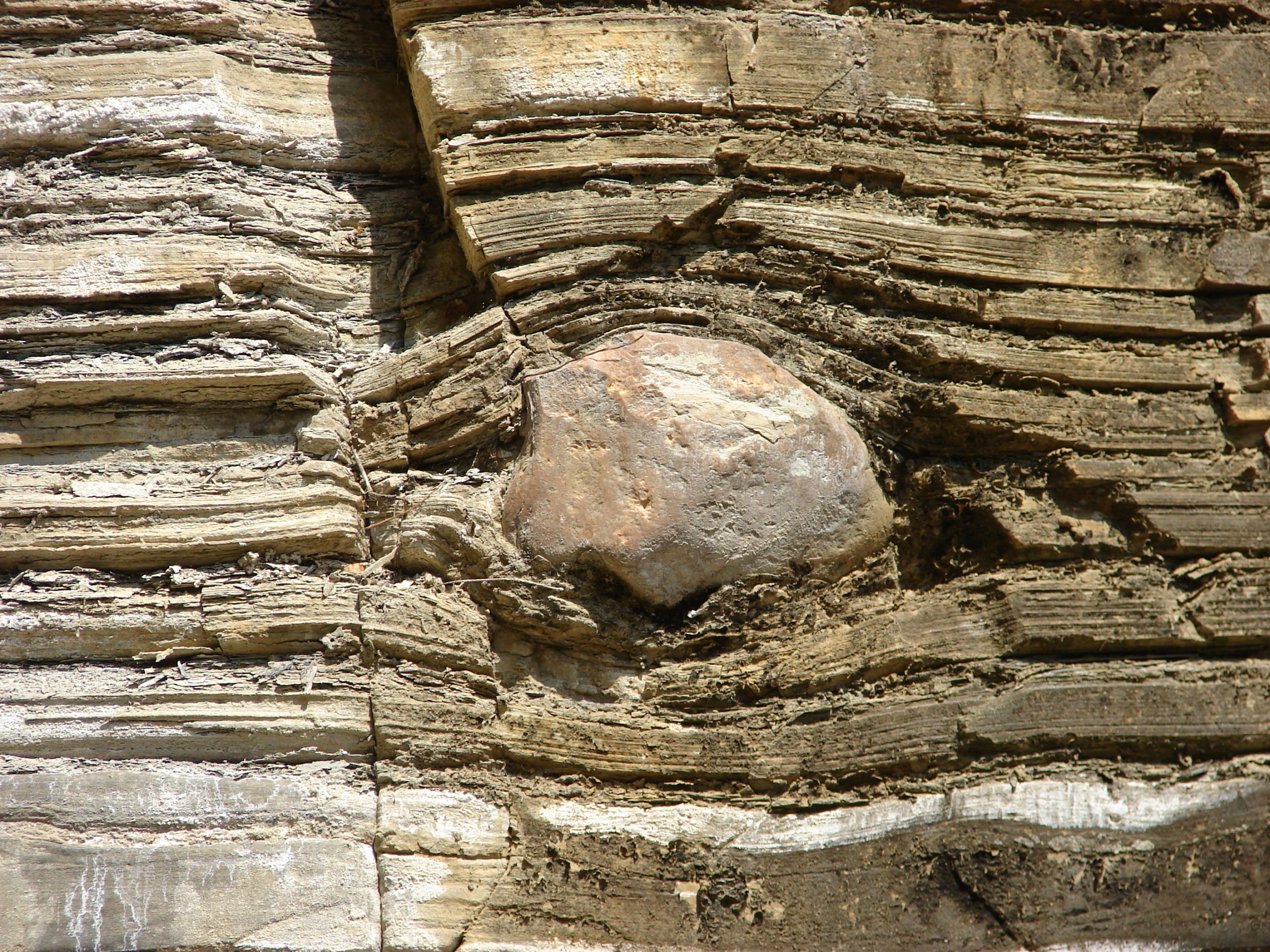
Blocks of floating ice sometimes occur on glacial lakes. When these melt they may drop larger blocks of rock into the otherwise fine-grained sediments on the lake-floor. Such dropstones are one of the most recognizable indications of glacial conditions in ancient fine-grained sediments. Dropstones deposited by melting icebergs, may also be found in glaciomarine sediments.
Permafrost
Landscapes around glaciers are described as periglacial[7]. A common feature of such landscapes is permafrost, frozen groundwater, that remains frozen for periods of 2 or more years. Permafrost underlies about 25% of the North American landscape, mainly in Canada’s north, but extending farther south in mountainous areas, and beneath the floor of the Arctic ocean in places.
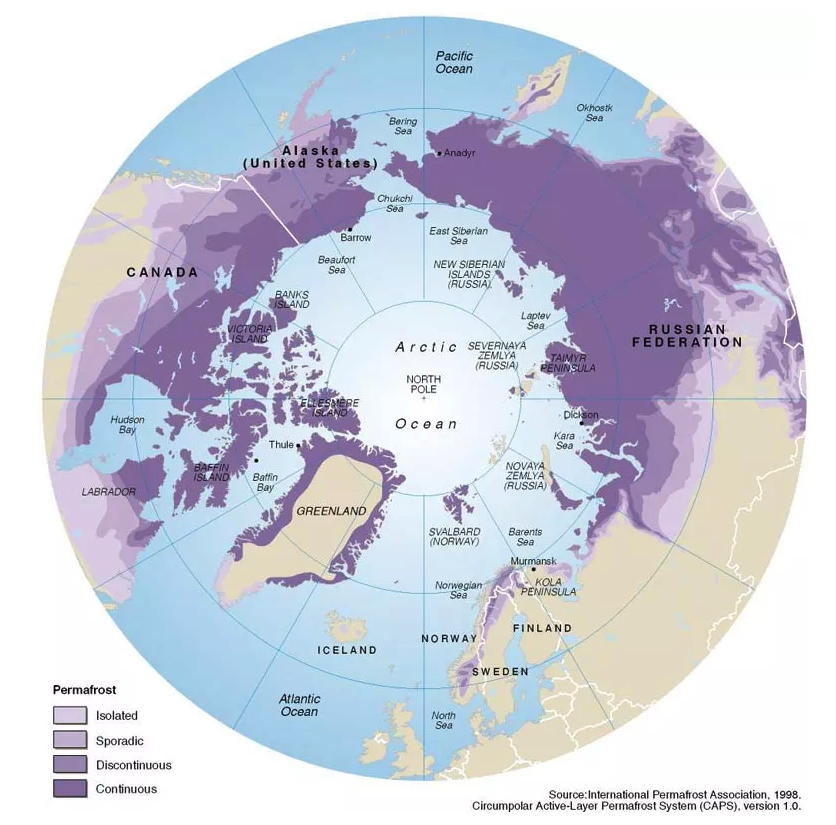
Traced downwards into the Geosphere, the permafrost layer eventually transitions into rock with liquid water in the pore spaces, because both increased temperature and pressure cause melting. In places, however, the permafrost layer may be over a kilometre thick.
In most areas with permafrost, an upper layer above the permafrost, known as the active layer, thaws every summer before refreezing each winter. The active layer typically ranges from 10 cm to ~2 m thick.
Periglacial landscape features
Several distinctive features of periglacial landscapes result from the interaction between water and permafrost.
Features of the active layer

Because the underlying permafrost is impermeable, water cannot easily escape from the active layer, so bogs and other wetlands are common. Construction of roads and buildings on the active layer requires special techniques. Ground that appears stable in the winter may turn into deep, wet mud during the summer, causing built structures to sink.
When the active layer is saturated and lies on a slope, it may undergo creep, producing solifluction lobes.
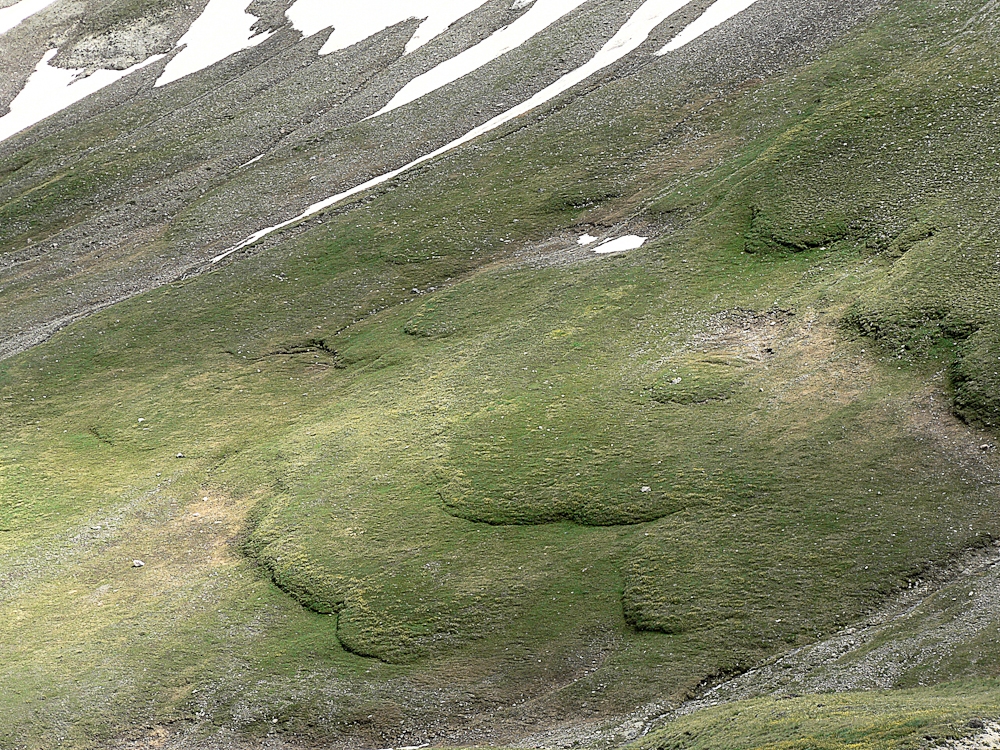
Ice wedges, patterned ground, and pingoes
Several distinctive features result from the expansion of water as it freezes to ice.
<diagram of ice-wedge formation>
During the late summer and autumn, the active layer may dry out where evaporation exceeds precipitation. The ground, including both the active layer and underlying permafrost, may crack as it dries and contracts. When water becomes available again it fills the cracks but may freeze to form a wedge of ice before the ground soaks up the water and returns to its original shape. The expansion of ice as it freezes may widen the crack, so that when the active layer melts again there may be space for more water; repeated freezing and thawing widens the crack and the wedge of ice it contains. Such ice wedges are common features of landscapes underlain by permafrost. Viewed from above they may produce a polygonal pattern known as patterened ground.
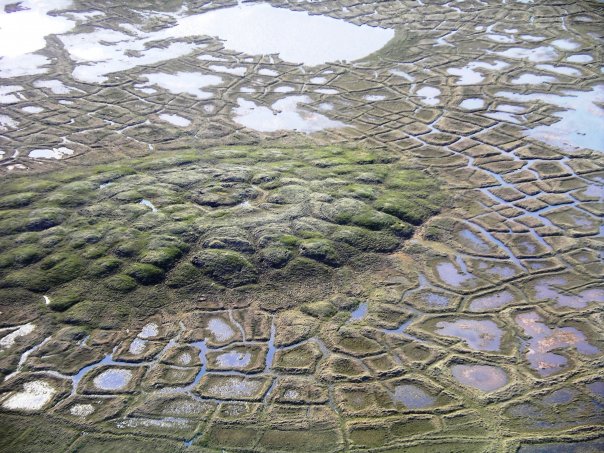

Pingoes are hills up to 90 m high and 800 m wide that are cored by ice. Pingoes typically form at sites of former lakes where liquid water was present at the surface. A lens of water is trapped when the surface freezes. Raising of the pingo occurs when this water is pressurized by either:
Importance of permafrost
In addition to containing ice and mineral material, permafrost stores a large amount of organic carbon. In contrast to warmer soils, the lack of liquid water and the cold temperatures present in permafrost inhibit natural microbial decay processes that convert organic matter back to carbon dioxide. (The same principle applies to storing food in a freezer!)
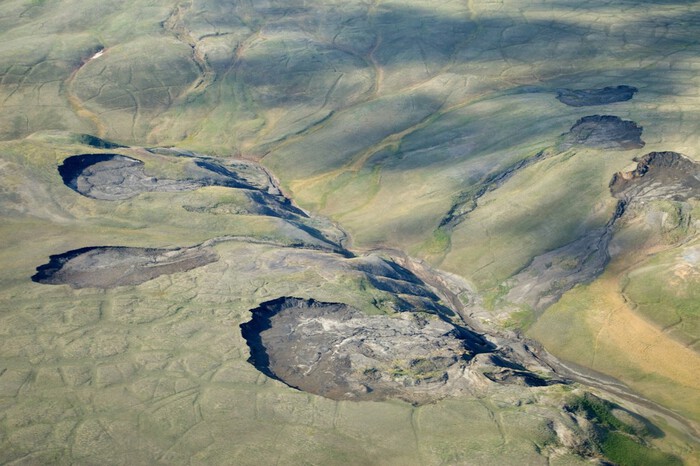
During periods of warming, permafrost returns stored carbon dioxide and methane to the atmosphere. Both gases are greenhouse gases that cause further warming of the atmosphere. The melting of permafrost therefore leads to positive feedback in the climate system.
In addition to this global impact, more local — but still important — impacts of warming are felt in areas with human populations that are underlain by permafrost. Buildings and roads may be affected by the rapid liquidization of ground that was formerly frozen, leading to disastrous subsidence. Slopes may become unstable, which can lead to rapid collapse of river banks and lake shorelines.
Sea ice
Sea ice is ice that has formed by freezing of the surface waters of the oceans. It should not be confused with the ice in ice shelves, which is derived from land ice that has flowed out onto the ocean surface.
Formation and distribution of sea ice
Sea ice begins to form as the air temperature falls below freezing point of seawater (-2°C) which is lower than that of fresh water because of its salinity). Initially small needles of ice form, known as frazil ice. Frazil ice crystals are typically 3 or 4 mm in diameter, and are nearly pure H2O in composition; the crystal lattice of ice is much less able to hold sodium and chloride ions in solution than liquid sea-water. The result is that the leftover water becomes more concentrated in sodium chloride (and other salts) than normal sea-water: it is a brine.
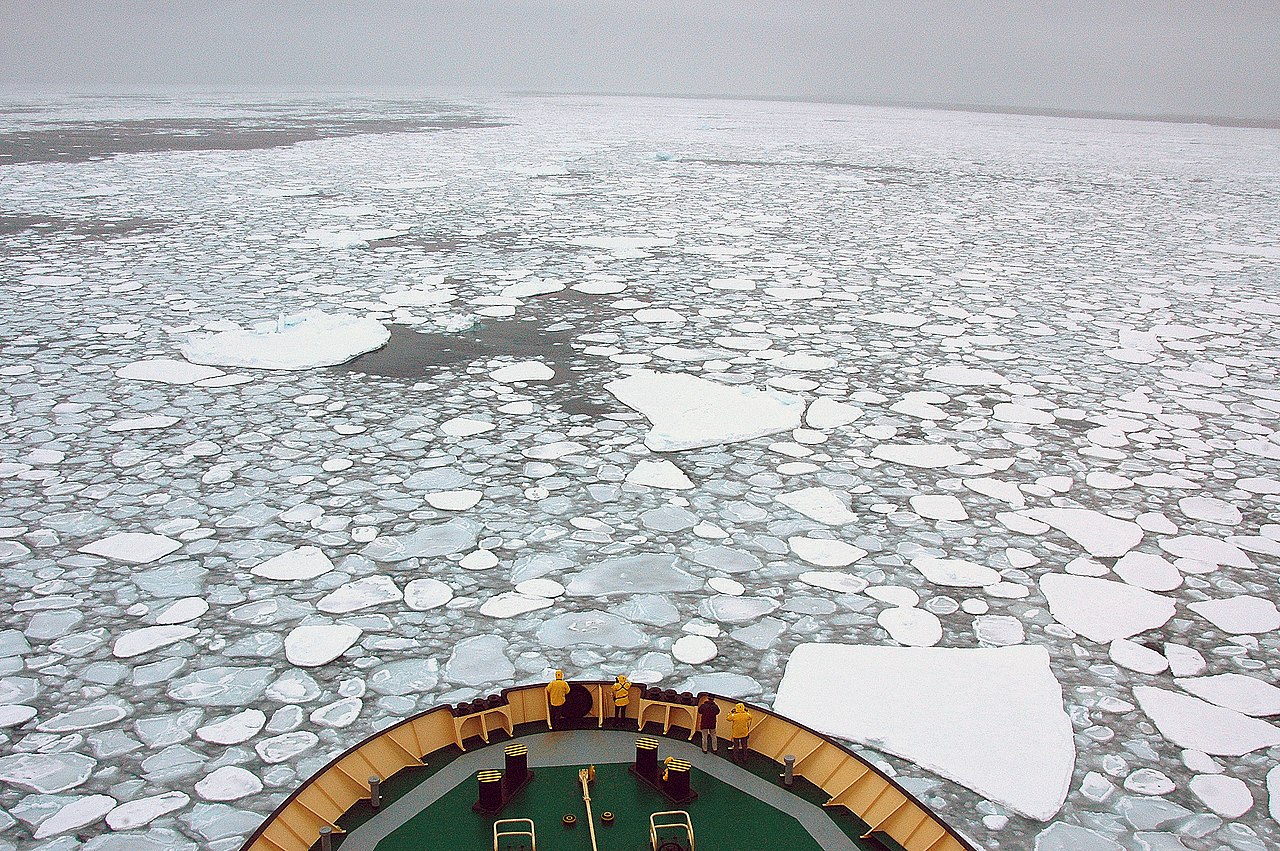
Eventually the frazil ice crystals freeze together. If the seA is calm, a continuous thin (< 10 cm) sheet of ice called nilas may form. If there is wave action, pancake ice, consisting of roughly oval pieces, may form. Eventually these join to form a continuous sheet, more than 10 cm thick, of pack ice. Drops of brine are initially trapped between the coalesced ice crystals, so sea ice initially contains some salt, though much less than normal sea-water. This salt tends to be expelled over time as the ice recrystallizes, so that multiyear ice has less salt than newly formed sea ice.
Subsequent freezing adds ice to to the base of the sea ice, so that in the first year it typically thickens to over 30 cm thick. Snowfall on sea ice, particularly in Antarctica, is also important. Multi-year ice may be up to 4 m thick in the Arctic Ocean. However, sublimation and melting typically remove ice from the surface. Therefore, a single ice crystal moves upward within pack ice over a period of time. Typically, the rate of this upward movement of ice is around 45 cm/yr.
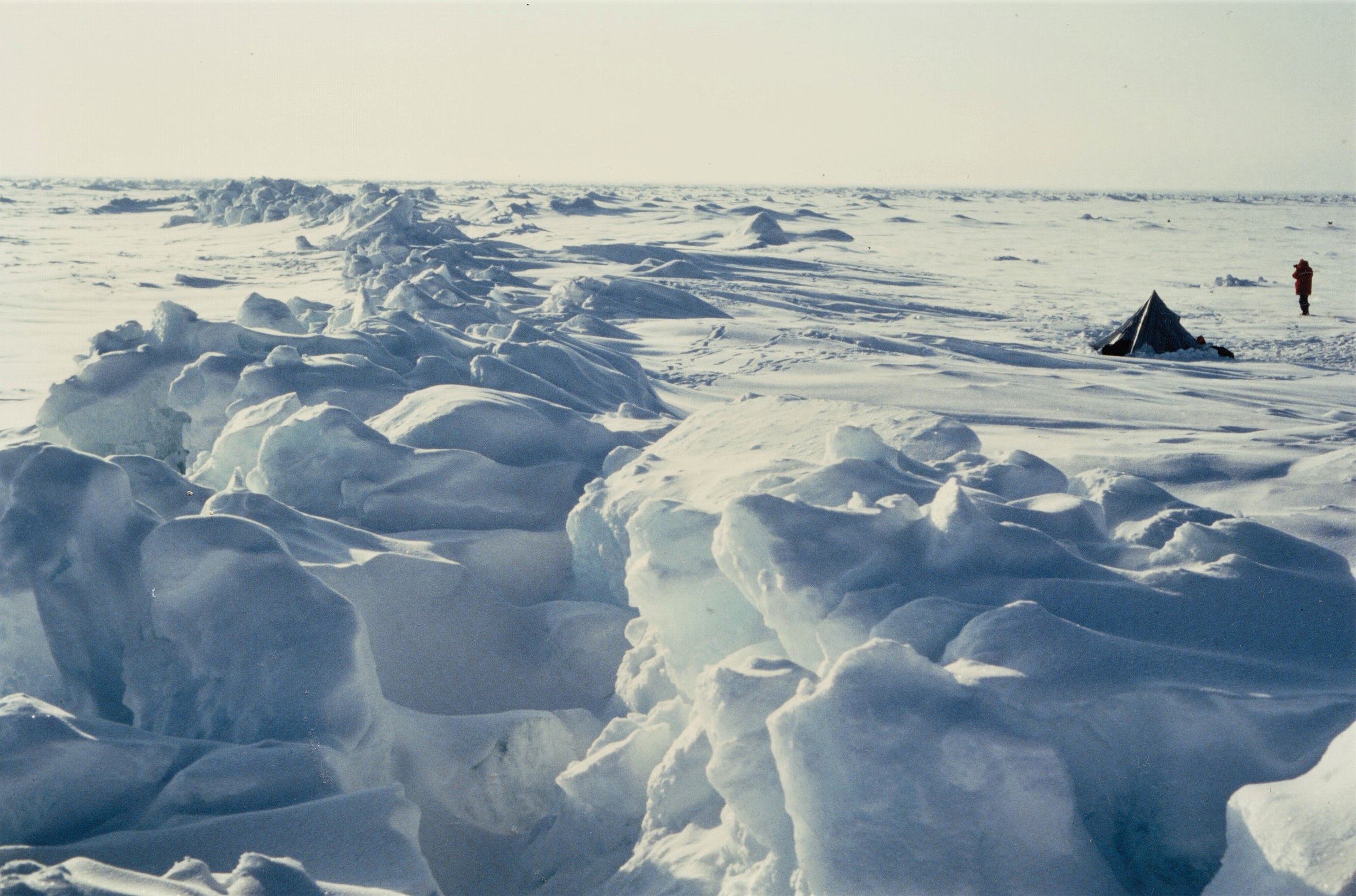
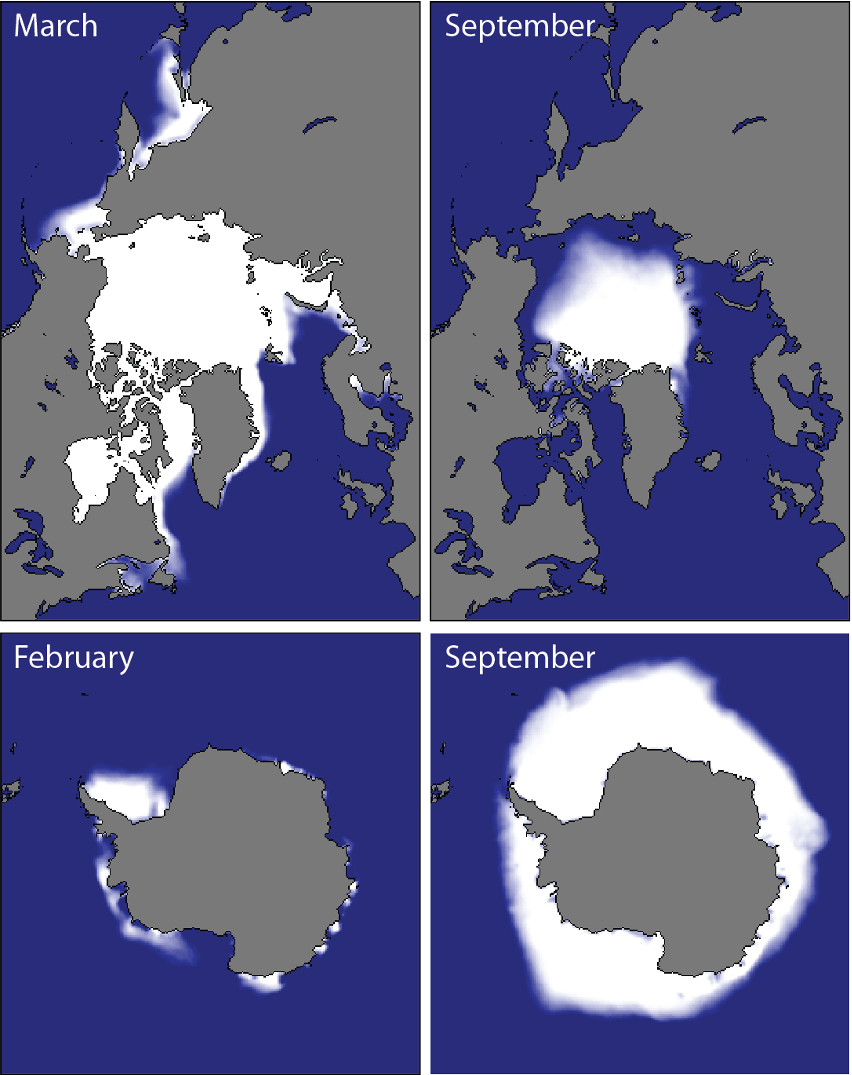
The Arctic Ocean is an ocean largely covered in sea ice. It has areas of perennial ice (i.e., multiyear) ice, but the both the area and the volume of Arctic sea ice have been declining since the beginning of accurate measurements.
Antarctic sea ice largely rings the continent Antarctica, but is largely seasonal — most of it melts every summer. The total amount of Antarctic sea ice has been relatively constant during the last four decades when Arctic ice was declining, but 2023 saw a very significant decrease in Antarctic sea ice.
Sea ice and Earth systems
Influence of sea ice
Although sea ice accounts for only about one tenth of one percent of the global ice volume, it has a disproportionate influence on Earth’s Atmophere and the rest of the Hydrosphere.
Sea ice is very reflective. The albedo of freshly formed sea ice is about 0.5, contrasting with the albedo of the open ocean surface at about 0.06. I thin layer of snow on the surface of sea ice may raise its albedo to 0.9 so that it reflects most of the incoming solar energy (both light and heat) back into space. Sea ice isolates the ocean — particularly the Arctic Ocean — from the atmosphere, limiting heat exchange between the two spheres where it occurs. Sea ice therefore contributes to the coldness of polar regions, helping to produce the steep temperature difference between the Equator and poles that drives atmospheric circulation. Lessening of this temperature gradient may be causing an increase in the amplitude of Rossby waves in the jet stream and associated stagnant pressure zones, leading to more intense storms, and periods of intense hot and cold weather in temperate latitudes.
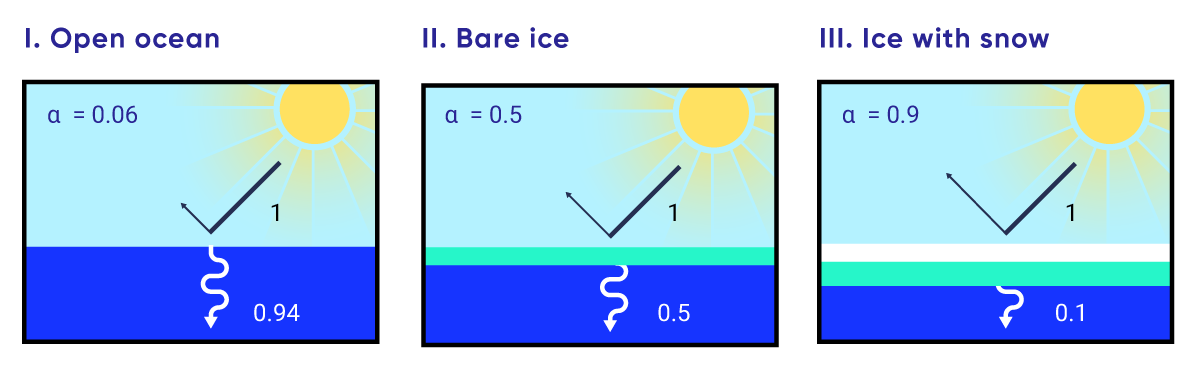
Sea ice is also an important contributor to ocean circulation. As sea ice freezes, salt is excluded, making the surface ocean water saltier. This cold, salty water is denser and sinks into deep ocean basins, driving thermohaline circulation in the deep ocean. Oceanographers refer to these streams of cold, salty water as the Antarctic bottom water (AABW) and the North Atlantic deep water (NADW). Changes in the amount of sea ice therefore have global consequences.
Recent changes in sea ice
Arctic
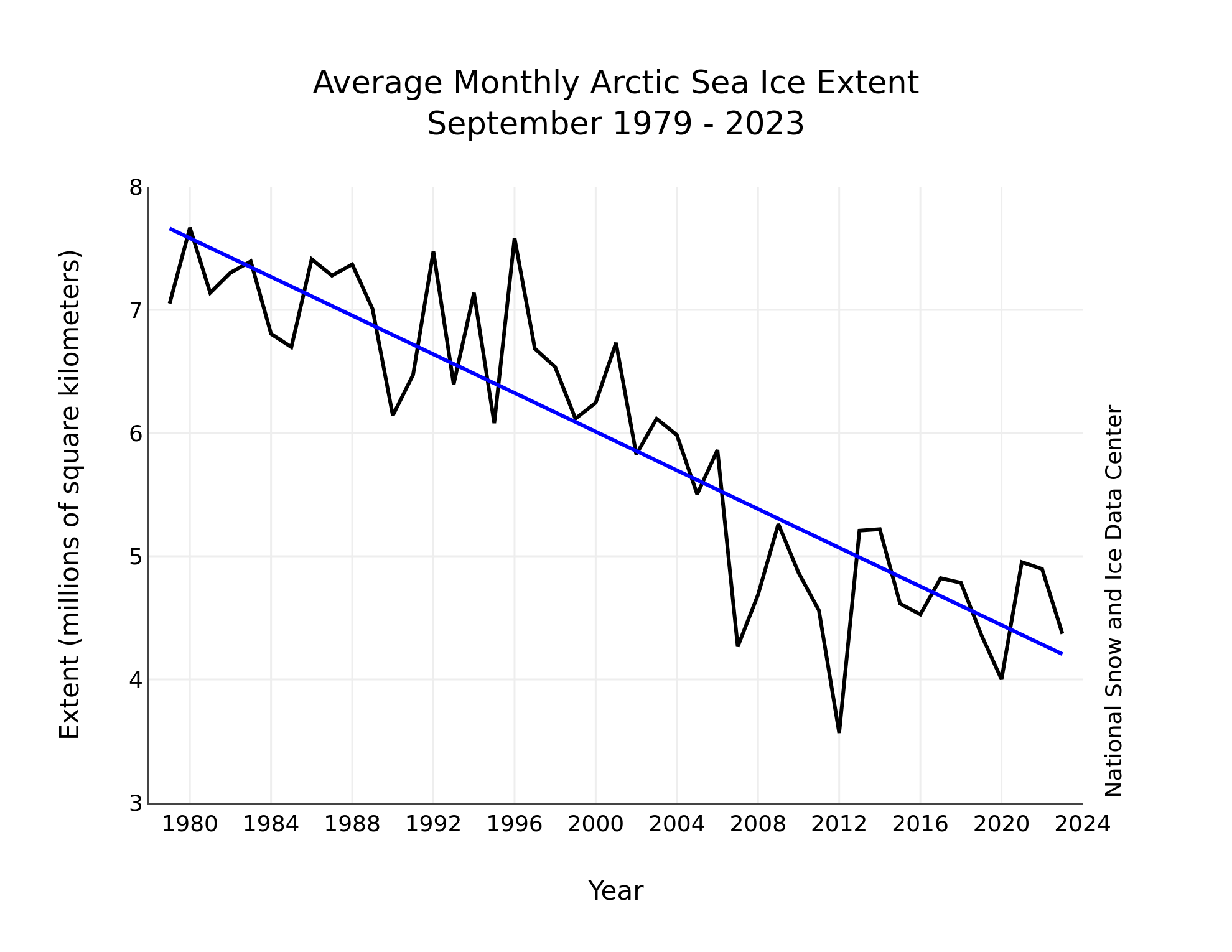
Satellite-based records of the area covered by Arctic ice using modern methods go back to about 1980. Many comparisons within that interval compare the amounts of Arctic sea ice in recent years with the average coverage from 1981–2010. During that interval Arctic sea ice typically reached a maximum extent during March (15–16 million km2) and fell to a minimum each September (around 6 x 106 km2), but the amount of Arctic sea ice has been clearly decreasing since 2000 CE. The lowest summer ice-cover occurred in 2012 (~3.5 x 106 km2). However, other years have shown other unusual characteristics. For example the ice amounts in 2020 were even lower than 2012 in certain months, while 2018 showed a winter ice coverage that was even lower than 2012. Overall, at the time of writing (August 2023) the 15 lowest sea-ice extents have all occurred in the last 15 years. The change has amounted to -3.2% per decade, though for late summer ice the change has been -11%.
The details can be explored in the interactive charts of the US National Snow and Ice Data Center.
There have also been changes in the overall age of the ice: the amount of multi-year ice, that is more resistant to melting, has decreased at 17% per decade, while the total ice volume has decreased at all times of the year
Antarctic
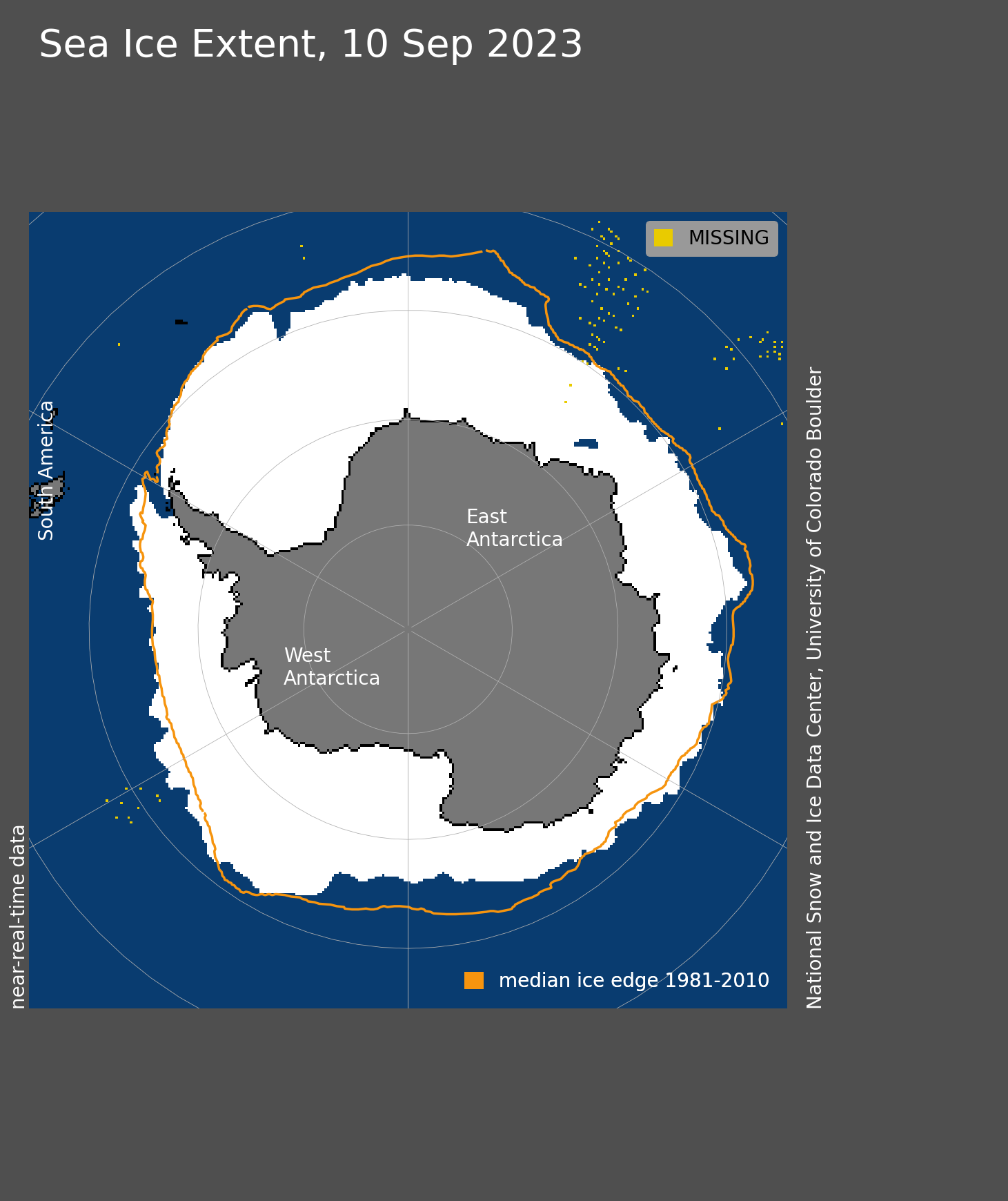
Antarctic sea ice is very different from Arctic sea ice, because it forms around a continent, rather in a small ocean basin that is largely surrounded by continents. Because of this distribution of land and sea areas, the Antarctic sea ice mostly forms at lower (warmer) latitudes so proportionally less survives summer melting and there is very little multi-year ice. As a result, the amount of sea ice is larger in Antarctic winter (September: 18.5 x 106 km2) but smaller in summer (February: ~3 x 106 km2) than the corresponding amounts in the Arctic. Antarctic sea ice is also thinner on average (mostly 1-2 m thick)
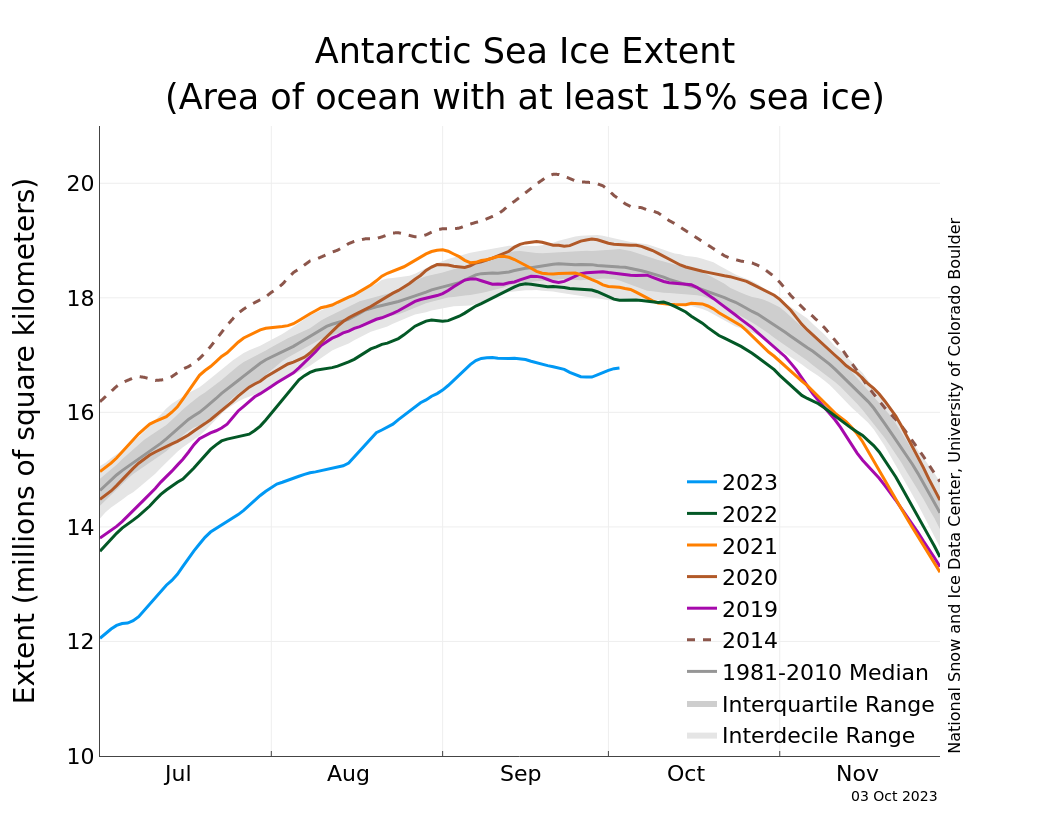
Overall there was a small increase in Antarctic sea-ice extent of ~1.5% per decade, over the interval that has seen a spectacular decrease in Arctic sea ice. However 2022 showed a lower extent of ice than any previous year, and at the time of writing, 2023 appears to be an exceptional year with 2 million square kilometres less ice than the 1981-2010 average.
Potential impacts of sea-ice loss
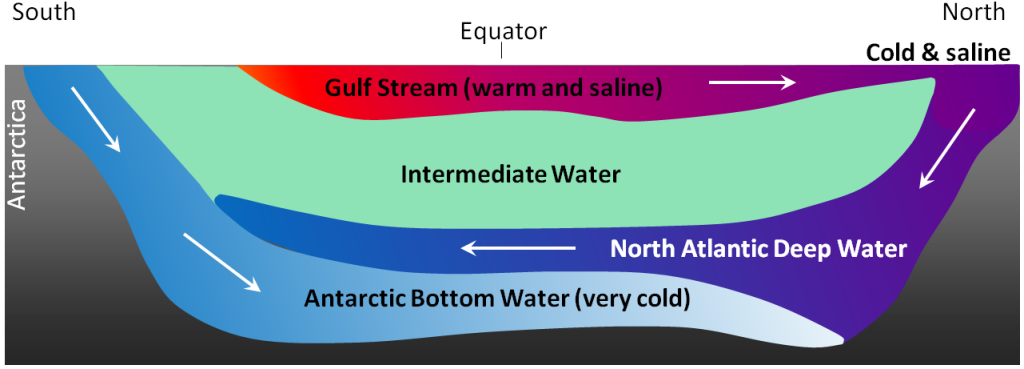
The potential effects of sea-ice loss are numerous.
- First, sea ice has a much higher albedo than open water. Hence loss of sea ice has a strong warming effect, causing a positive feedback loop in the climate system.
- The formation of Arctic sea helps to form cold salty water that sinks as North Atlantic deep water (NADW). As sea ice shrinks, ocean waters become less salty and warmer, slowing the flow of NADW[9] with potentially large (and largely unknown) implications for ocean circulation.
- Loss of sea ice in recent decades has contribued to polar amplification of warming: latitudes near the poles experience greater warming than equatorial regions.
- Sea ice also insulates the ocean from heat loss (or gain) and provides a barrier to gas exchanges between the ocean and Atmosphere.
- Finally, sea ice is major component of polar ecosystems. Many marine mammals, in particular, depend on sea ice to feed and breed. Loss of sea ice may have a major impact on species such as polar bears, seals and walrus.
These effects are further explored in the chapter on climate and climate change.
- "Snowline" may alternatively be written "snow line" ↵
- The accumulation zone is also known as the "zone of accumulation" ↵
- Ablation zone or "zone of ablation" ↵
- This was observed following the collapse of the Antarctic Peninsula's Larsen B Ice Shelf. ↵
- This is known as the principle of floatation, itself a consequence of Archimedes' principle: the buoyancy force on an object in a fluid is equal to the weight of fluid displaced. Therefore, a floating object displaces exactly its own weight of water. ↵
- Note that the asymmetry of drumlins is opposite to the asymmetry of roches moutonnées, which are made of solid rock and are produced entirely by erosion, not deposition. ↵
- From the Greek "peri", meaning around ↵
- The two types of pingo described here are known, respectively, as closed system pingos and open system pingos. ↵
- Signs of a slowdown are now (2023) being observed. ↵
A system containing all Earth's ice
A molecule with distinct positive and negative electric charges on either end
The proportion of light that is reflected from the surface of a substance
Ice crystals formed by condensation directly from water vapour, that fall as precipitation
Masses of ice capable of slow movement over the land surface
Ice that has formed on the sea surface by freezing of seawater
Solid Earth material with pore spaces filled by ice
Material near the Geosphere surface that remains frozen for two or more years.
A cycle in which the products of a process act as inputs increasing the activity of the same process
The level on a glacier, or more generally on any sloping land surface, above which snow persists year-round
Close to either the North Pole or the South Pole
A period of Earth history during which large areas are covered by glaciers
The Earth’s climatic state during times of cyclic expansion and retreat of ice sheets.
A warm state of Earth’s climate, without continental ice sheets.
Partially compacted snow in its first year.
Ice particles formed by the loss of air in snow that has been resting on the surface of the Earth for more than a year
Describes a material or process of deformation in which strength is suddenly lost across a fracture plane or planes
Continuous deformation of crystalline materials that takes place after a yield stress is exceeded
Gain of ice in a glacier
The lower limit of a glacier
The movement of the terminus of a glacier in the same direction as ice flow, so that more land is progressively covered by ice; Typically occurs when accumulation exceeds ablation.
The movement of the terminus of a glacier in the opposite direction to ice flow, so that less land is progressively covered by ice; Typically occurs when ablation exceeds accumulation.
An exposed rock mountain top projecting through an ice sheet or ice cap
A lake hidden below glacier ice
A floating extension of a land-based glacier
The line on the sea-floor between glacier ice that is floating and glacier ice that is resting on the bottom
The break-off of icebergs from glacier ice that is at sea level
Sheet-like; applied to flow of fluids, it describes flow along sub-parallel lines, lacking the gyres typical of turblence.
The physical breakdown of rock into smaller particles at the surface of the Geosphere.
The mechanical weathering effect of water in thin cracks as it freezes to form ice, splitting rocks apart as a result of expansion.
Glacial plucking describes a process where a moving glacier removes bedrock fragments that have become surrounded by ice
Physical weathering caused by contact between moving rock fragments and larger rock surfaces
Grooves carved in bedrock as a result of glacier movement
Short notches, transverse to movement direction, found on glacially eroded or faulted surfaces
Rock affected by glacial erosion that has a smooth upstream side due to abrasion and a rugged downstream side due to plucking
A small glacial valley that enters a larger glacial valley high on the side of the larger valley
A bowl-shaped glacial valley that is approximately as short as it is wide (equant)
A jagged ridge between two glacial valleys
A jagged peak formed in glaciated terrain where three or more arêtes meet
A channel carved by a sudden release of glacial meltwater
Unsorted sediments deposited directly from glacier ice
The presence of layers at centimetre or larger scale, having distinct composition an/or texture, typical of sedimentary rocks
Distinctive landscape shapes characteristic of particular Earth-surface processes
An accumulation of glacial till
An accumulation of till or rock fragments along the edge of an Alpine glacier
Processes that move dense solid material down slopes on the surface of the Geosphere due to the force of gravity.
A linear accumulation of till in the middle of an Alpine glaciar, formed by the merging of two lateral moraines
A moraine formed at the terminus of a glacier.
A body of till marking the furthest extent of a glacier’s ice.
Bodies of till left by successive positions of a glacier terminus during glacier retreat of a glacier.
A tear-drop-shaped hill, a subglacial landform formed by ice sheets moulding subglacial sediment
Formed below a glacier
A block of rock that has been moved by a glacier so that it appears foreign to the bedrock that it rests on
Describes environments with rivers derived from melting of glacier ice
Describes lake environments associated with glaciers
Material composed of numerous rock fragments, as used by humans in construction
Sediment carried along the water-bottom (usually of a river) through rolling, sliding, and saltation
Having approximately equal length and width
A glacial landform made of sand or gravel deposited by water flowing along the edge of a glacier
Braided streams have multiple branches that branch and rejoin
River behaviour characterized by large changes in channel direction (bends).
Formed in the environment immediately outside the terminus of a glacier
A lake or depression in a periglacial area, typically formed as a result of the melting of a stranded ice block during glacier retreat
A time about 20 thousand years ago when glaciers reached a maximum extent.
Lamina (plural laminae): a thin layer (less than 1 cm thick)
Alternating layers deposited in lakes, representing annual glacial melt events.
A clast of rock that has fallen from floating ice into sediment below
Describes environments in the sea that are influcence by nearby glacier ice.
A form of sea ice consisting of separate needles, typically 3–4 mm long, floating in water
A stage in the development of sea ice, characterized by roughly oval ice masses 30 cm – 3 m in diameter
Sea ice that covers more than 70% of the sea surface with slabs metres to kilometres across
Sea ice that has lasted more than one year
A positive feedback phenomenon whereby more of the effect of climate change is felt in Arctic or Antarctic latitudes than is felt at the equator.